Abstract
The objective of this study was to track the changes and the relationship between structure and functional properties of rabbit myosin during heat-induced gelation, and to provide more insight into the gelation properties of rabbit myosin. Storage modulus (G′) and loss modulus (G′′) during the heat-induced gelation process were measured by a rheometer, and the water holding capacity (WHC) and hardness of the heat-induced gel were measured by centrifugation method and texture analyzer, respectively. The quantitative analysis of secondary structural content was performed by circular dichroism (CD). The CD data were fitted to three secondary structures, which were α-helix, β-sheet, and random coil. The results showed that the WHC, hardness, G′, G′′, β-sheet, and random coil increased with the increase of temperature, while the α-helix decreased. The changes of structural properties of myosin determined the functional properties during the heat-induced gelation process, and they had a very strong correlation.
El objetivo de este estudio consistió en dar seguimiento a los cambios ocurridos durante la gelificación inducida por calor y a la relación existente entre la estructura y las propiedades funcionales de la miosina de conejo durante dicho proceso. Asimismo, el estudio pretendió generar más información acerca de las propiedades de gelificación de la miosina de conejo. En el transcurso del proceso de gelificación inducida por calor, se midieron el módulo de almacenamiento (G′) y el módulo de pérdida (G′′) mediante el uso del reómetro; mientras que, la capacidad de retención de agua (WHC) y la dureza del gel inducida por el calor, fueron medidas empleando el método de centrifugación y el analizador de textura, respectivamente. El análisis cuantitativo del contenido estructural secundario fue realizado mediante dicroísmo circular (CD). Los datos obtenidos a partir del CD se aplicaron a tres estructuras secundarias: hélice α, lámina β, y espiral al azar (random coil). Los resultados demostraron que cuando se incrementó la temperatura, la WHC, la dureza, G′, G′′, la lámina β y la espiral al azar aumentaron, mientras que la hélice α disminuyó. Los cambios experimentados por las propiedades estructurales de la miosina determinaron las propiedades funcionales durante el proceso de gelificación inducida por calor, demostrando, además, un grado de correlación muy alto.
1. Introduction
In meat processing and production, salt-soluble proteins form a three-dimensional protein gel network, which affects the water holding capacity (WHC), oil holding capacity, viscoelasticity, textural properties, and the sensory aspects of meat products (Xiong, Citation1997). Many studies have reported that myosin, the major muscle protein, plays a major role in the formation of heat-induced gels (Hermansson, Harbitz, & Langton, Citation1986; Sano, Noguchi, Matsumoto, & Tsuchiya, Citation1990; Yamamoto, Citation1990). Heating leads to myosin denaturation and aggregation, eventually forming a three-dimensional gel network. The molecular transition of the protein from its native state to the denatured state involves conformational changes in the quaternary, tertiary, and secondary structures such as α-helix, β-sheet, β-turn, and random coil contents (Ker & Toledo, Citation1992), which indicate that there may be some correlations between structures and gel properties such as WHC, textural properties and rheological properties (Liu, Zhao, Xiong, Xie, & Qin, Citation2008).
Rheological properties of substances are the mechanical properties (physical stress and other factors) that occur during deformation or movement (Xiong, Citation1997). These mechanical properties are related with the composition of molecules, combinations, and dispersions of intra- and inter-molecular and microstructures of the gel. Fukushima et al. (Citation2005) studied the changes of pollack and white croaker myosin storage modulus G′ and proposed the gel formation mechanism of fish myosin. And gaining an understanding of the gelation properties of myosin and meat gel systems is beneficial for the development of processed meat products as well as maintaining quality of meat products (Feng & Hultin, Citation2001).
Circular dichroism (CD) uses the difference between left and right circularly polarized light absorbance of optically active substances to study the structural information of biological macromolecules (Choi & Ma, Citation2007). Typical α-helical structures show two negative bands in the CD 222 nm and 208 nm, while β-sheet structures appear a positive absorption peak at 198 nm (Greenfield, Citation1996). Using CD, King, Seidel, and Lehrer (Citation1995) found two spiral unfolding cooperative transitions at 222 nm; a minor transition at 46°C representing unfolding of the subfragment, S-2 region and another at 58°C representing unfolding of the tail light meromyosin (LMM). Temperature also plays a significant role during the gelation of myosin, which affects the unfolding and aggregation of myosin molecules (Xiong & Brekke, Citation1990). Until now, the relationship between the changes in structure and functional properties of myosin during heat-induced gelation has been an almost unexplored area. This study used myosin prepared from rabbit skeletal muscle to investigate temperature as a factor to study the changes in the secondary structure using CD, and to estimate its relationship with WHC, gel strength, and rheological properties, to provide a theoretical base for improved meat products.
2. Materials and methods
2.1. Materials
The meat used in the present study was rabbit skeletal psoas (Psoas major, PM) from a stud rabbit breeding farm (Academy of Agricultural Sciences of Jiangsu Province, China). The muscle was immediately chilled 24 h postmortem, frozen after that and stored at –20°C until required for the extraction of myosin. Ethylene glycol bis(beta-aminoethyl ether)-N,N,N’,N’-tetraacetic acid (EGTA) was obtained from Sigma Chemical Co (Louis, USA). Triton X-100 was purchased from Amersco (San Francisco, USA). All other chemicals used in this work were also from commercial sources and of analytical grade.
2.2. Extraction of myosin and preparation of gel system
Extraction of myosin was carried out essentially as described by Han, Zhang, Fei, Xu, and Zhou (Citation2009), with some modifications according to the protocol of Wang and Smith (Citation1994). Briefly, trimmed, ground muscle (about 50 g) was washed and homogenized using four volumes of a post-rigor extraction buffer (0.1 mol/L Tris,10 mmol/L EDTA, pH 8.3) in a Waring Blender, Model No. 8010ES (Waring Commercial, New Hartford, CT). Samples were then centrifuged 1000×g for 20 min (Beckman Avanti J-E centrifuge, Beckman Coulter, Fullerton, CA, USA). The pellet containing myosin was then resuspended and washed three times in four volumes of standard salt solution (SSS) (0.1 mol/L KCl, 20 mmol/L K2HPO4/KH2PO4, 2 mmol/L MgCl2,1 mmol/L EGTA, 1 mmol/L NaN3, pH 7.0), and then homogenized using a Ultra Turrax T25 BASIS (IKA Labortechnik, Germany). Centrifugation (1000×g for 10 min) was used to collect the pellet between washes. The pellet was resuspended and washed twice in four volumes of SSS +1% Triton X-100 and centrifuged (1500×g for 10 min). The pellet was then resuspended and washed two additional times in three volumes of solution A (0.15 mol/L K2HPO4/KH2PO4, pH 7.0) and centrifuged (10,000×g for 10 min). The pellet was resuspended and washed twice in three volumes of G-S extraction solution (0.3 mmol/L KCl, 0.15 mol/L K2HPO4/KH2PO4, 5 mmol/L MgCl2, 1 mmol/L EGTA, 5 mmol/L NaPPi, 2 mmol/L ATPNa2, pH 6.5) and stirred for 20 min, then centrifuged (5000×g for 10 min) and the supernatant was collected. Nine volumes of precooled distilled water were added to the supernatant and then centrifuged (12,000×g for 12 min). The pellet was then resuspended and washed in three volumes of solution B (0.1 mol/L KCl, pH 7.0) and again centrifuged (1,500×g for 10 min). The pellet was resuspended and washed in solution C (0.6 mol/L KCl, 0.15 mol/L K2HPO4/KH2PO4, 5 mmol/L MgCl2, 1 mmol/L EGTA, 5 mmol/L NaPPi, 2 mmol/L ATPNa2, pH 6.5) and stirred for 30 min. Pre-ground (NH4)2SO4 was added into the solution to reach 35% saturation, then centrifuged (10,000×g for 25 min) and the supernatant was collected. Ammonium sulfate was again added to the solution to achieve 48% saturation and then stirred for 20 min. It was then centrifuged (10,000×g for 25 min) and the pellet was collected and resuspended and washed in one volume of phosphate buffered saline (0.6 mol/L KCl, 20 mmol/L K2HPO4/KH2PO4, 1 mmol/L EDTA, pH 6.5). The mixing was dialyzed for 24 h with phosphate buffered saline (0.6 mol/L KCl, 20 mmol/L K2HPO4/KH2PO4, 1 mmol/L EDTA, pH 6.5), and changed every 6 h. The purified myosin was kept at 0–4°C and each preparation was used within less than a week.
For preparation of myosin gels, myosin was diluted to 10 mg/mL and about 2 mL myosin was transferred to a 5 mL capped plastic centrifuge tube. The tubes were heated at a rate of about 1°C/min from 20°C to 90°C (with the center temperature reaching 20°C, 30°C, 40°C, 50°C, 60°C, 70°C, 80°C, and 90 °C), which was performed using a water bath and held for 20 min after the destined temperature reached. Gels were kept at 0–4°C overnight (12 h) for measurement of WHC and texture characteristics.
2.3. Measurement of WHC and texture characteristics
WHC (%) was determined according to the method developed by Kocher and Foegeding (Citation1993). Gels were centrifuged at 10,000×g for 10 min (Beckman Avanti J-E centrifuge, Beckman Coulter, Fullerton, CA, USA) at 4°C. The weights (g) of the centrifuge tubes, protein samples, and moisture loss were all recorded. The following formula was used to determine WHC (%):
where ML is the amount (g) of moisture lost from the gel during centrifugation and CG is the weight (g) of the heated gel. Each measurement was performed in triplicate.
Gel hardness was measured by a TA-XT 2i SMS Stable Microsystems Texture Analyser (Stable Microsystems Ltd., Godalming, Surrey, England) with the Texture Expert program using the texture profile analysis (TPA) protocol. Force–time deformation curves were obtained with a 25 kg load cell applied at a cross-head speed of 0.5 mm/s. Texture analysis parameter settings were: pre-test probe (P5 5 mm diameter cylinder stainless) rate of decline was 2.0 mm/s, test speed 1.0 mm/s, after test speed 1.0 mm/s, puncture test distance 5 mm, and trigger force 3 g. The textural parameter was measured according to Bourne (Citation1978): hardness (g), the maximum force required to compress the sample was recorded. Each measurement was performed in triplicate.
2.4. Test of rheological properties
Rheological properties of prepared myosin (10 mg/mL) were measured during heat-induced gelation using a Physica MCR301 rheometer (Anton Paar, Austria) in small strain oscillatory mode. Measurements were conducted within the linear range at a strain of 0.02, and a constant frequency of 0.1 Hz. Samples were heated from 20°C to 90°C at a rate of 1°C/min between 50 mm parallel plates (gap=0.5 mm). The exposed surfaces of the protein samples were coated with a liquid paraffin to prevent drying. The storage modulus (G′) and loss modulus (G′′) were measured during linear heating (Westphalen, Briggs, & Lonergan, Citation2005). Each measurement was performed in triplicate.
2.5. Test of CD
CD was carried out in the Core Facility Center for Life Sciences, University of Science and Technology of China. The CD spectrum was measured using a Jasco J-8l0 spectropolarimeter (Jasco Co. Ltd., Tokyo, Japan). The myosin sample (0.1 mg/mL) was transferred to a quartz cell with a 1 cm light-path length. Molecular ellipticity was measured in the range from 190 to 250 nm, the scan rate was 100 nm/min, and the temperature was regulated with a control unit. The temperature was increased at a rate of 1°C/min from 20°C to 90°C. The secondary structure percentage of myosin was calculated using information from DichroWeb website (http://dichroweb.cryst.bbk.ac.uklhtml/home.shtml) (Whitmore & Wallace, Citation2004), the algorithm was K2D (Andrade, Chacón, Merelo, & Morán, Citation1993) with the reference protein SP175 and the mean residue weight of myosin was assumed 110 g/mol. Each measurement was performed in triplicate.
2.6. Statistical analysis
Statistical analysis of results was performed using Statistical Analysis System (SAS 8.12, SAS Institute Inc., Cary, NC). Analysis of variance (ANOVA) was employed to determine the significance of main effects. Significant differences (P < 0.05) between means were identified using Duncan’s multiple range test.
3. Results and discussion
3.1. WHC and Hardness of Myosin Gel
The gelation process of myosin transforming into a three-dimensional network structure can be described in three stages: denaturation, aggregation, and cross-linking (Maltais, Remondetto, & Subirade, Citation2008). When the three-dimensional gel network formed, the gel appeared as a dense mesh, holding a large amount of capillary-bound and chemically bound water. Hermansson and Buchheim (Citation1981) inferred that this capillary-bound water played an important role in the WHC of the gel, and was affected by the pore size and integrity of the network structure. At temperatures of 20°C and 30°C, myosin aggregation did not occur and myosin remained in the solution; therefore, the WHC was 0 after centrifugation. As shown in , the WHC of myosin increased significantly (P < 0.05) from 0 to 66.03% ± 1.69% as temperature increased from 30°C to 50°C; however, no further increase was observed (P > 0.05) with the increase of temperature from 50°C to 80°C. However, as the temperature continuously increased to 90°C, the WHC of myosin decreased significantly (P < 0.05) to 57.02% ± 1.18%, probably because the prolonged high temperature lead to shrinkage of myosin.
Figure 1. Effect of temperature on the water holding capacity (WHC) and hardness of myosin gels.
Figura 1. Efecto de la temperatura en la capacidad de retención de agua (WHC) y en la dureza de geles de miosina.
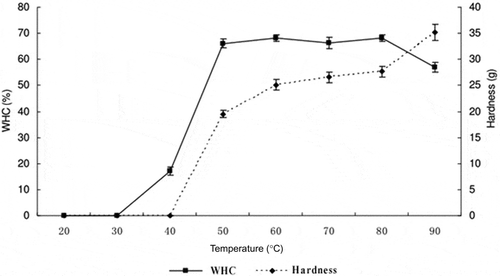
During the heat-induced gelation process, hardness followed a trend similar to that of WHC. In the test for gel hardness, myosin solution can only be measured with the texture analyzer when the temperature reaches 50°C, so the hardness was 0 below 50°C. Gel hardness then significantly (P < 0.05) increased from 50°C to 80°C; however, the rate of increase decreased. Beyond 80°C, gel hardness increased significantly (P < 0.05) to 35.14 ± 1.58 g, possibly because the gel shrunk and lost water.
From the trend of WHC and hardness during heating, we could infer that myosin aggregated at about 40°C. As the temperature increased, the aggregation became greater and formed a three-dimensional network. The formation of network increased the hardness, while water filled between pores by porous capillary action, which led to the increase of WHC. However, as temperature increased to 80°C with continued heating, the network began to be destroyed and capillary water came out from the network structure, which resulted in the decrease of WHC and increase of hardness.
3.2. Rheological properties
shows the changes of storage modulus and loss modulus values of myosin during heating. From 20°C to 40°C, the storage modulus value of myosin remained stable, indicating that the protein molecules were not undergoing aggregation. However, the storage modulus value had a rapid increase in the range 42–53°C, and then the increase rate became slow from 54°C to 60°C. The storage modulus value continued to increase to 80°C, and did not change significantly beyond 80°C. The loss modulus (G′′) value showed similar trends to storage modulus (G′). The initial change temperature of loss modulus value was 45°C. The increasing rate reduced profoundly at 55°C. The loss modulus value remained stable after 70°C.
Figure 2. Effect of temperature on G′ and G′′ of myosin gels.
Figura 2. Efecto de la temperatura en G′ y G′′ de los geles de miosina.
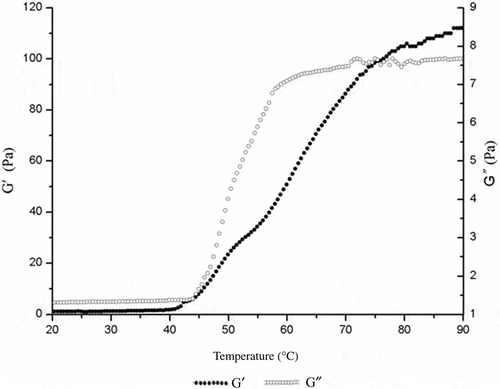
Xiong and Blanchard (Citation1994) found that the myofibrillar protein storage modulus value changed at 46°C during heating, and Samejima, Ishioroshi, and Yasui (Citation1981) found that rabbit myosin had two elastic transition points; one at 43°C and another at 53°C, which is similar to the findings of the present research. The storage modulus and loss modulus values of gel were stable when the temperature rose to about 70°C, which is consistent with the results of myofibrillar proteins (Westphalen, Briggs, & Lonergan, Citation2006). This suggested that the storage and loss moduli during heating were like “S” shape, which were stable at first and increased sharply in the middle, then flattened at the end.
3.3. CD of myosin
The results of CD measurement of myosin during heating are shown in . At 20°C, in the range of 250–200 nm wavelength, two negative peaks appeared at 208 and 222 nm, which indicated typically high α-helical content (Johnson, Citation1988). As the temperature increased molar ellipticity of the two negative peaks was significantly decreased, which showed the loss of α-helical structure and possible formation of other secondary structures (Liu et al., Citation2008). shows the secondary structure content of myosin at different temperatures. The content of α-helix between 20°C and 40°C did not significantly change and was stable at around 82%. This indicated that the myosin rod containing the rich α-helical structure was not involved in the formation of gel below 40°C (King et al., Citation1995). The content however decreased immediately from 40°C to 70°C and remained stable at 29.67% beyond 70°C. The content changes of α-helix showed the destruction of myosin rod after 40°C and the exposure of groups of amino acids to the surface and involved in the formation of the gel.
Figure 3. Effect of temperature on the circular dichroism (CD) spectra of myosin gels. a: 20°C; b: 30°C; c: 40°C; d: 50°C; e: 60°C; f: 70°C; g: 80°C; h: 90°C.
Figura 3. Efecto de la temperatura en el espectro del dicroísmo circular (CD) de los geles de miosina.
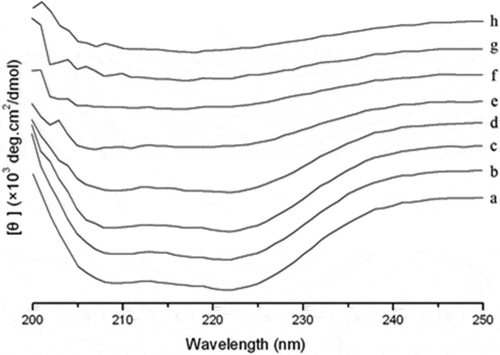
Figure 4. Effect of temperature on the secondary structure of myosin gel.
Figura 4. Efecto de la temperatura en la estructura secundaria del gel de miosina.
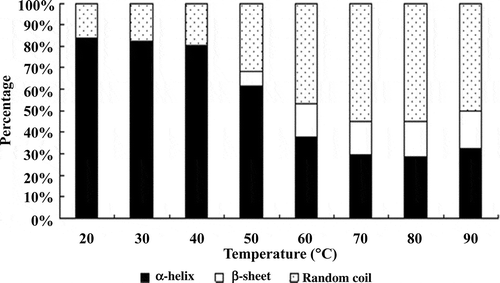
On the whole, the content of β-sheet was increased as temperature increased from 20°C to 90°C. In the region of 40°C to 60°C, there was a sharp change in area, from about 0 to about 15%. The content of β-sheet only increased a little at temperatures above 60°C, but these increases were not significant. The content of random coil increased during heating, and the increase in area was mainly in the range of 40°C to 80°C.
A large number of studies have found the general phenomenon of decreased α-helix and increased β-sheet and random coil during heat-induced process of myosin gel formation (Li-Chan & Nakai, Citation1991). Liu et al. (Citation2008) studied the changes in the secondary structure of porcine myosin from 5°C to 95°C, and found that α-helix content decreased from 90% to 40% with the increase of temperature, while β-sheet content increased in a nonlinear manner; the process of heat-induced gelation of porcine myofibrillar proteins also followed a similar manner (Xu, Han, Fei, & Zhou, Citation2011), which was consistent with our study. Compared with the myosin head region, which has rich sulfhydryl content, the myosin tails have high α-helix structure. Due to the temperature, the myosin tail region may be destroyed and LMM may deform, which causes the loss of α-helix. Then, the hydrophobic groups buried in the intermolecular myosin tail regions interact with other adjacent groups and promote the formation of the network. It has been shown that the loss of α-helix is closely related to the unfolding of myosin through the study of different types of fish myosin (Chan, Gill, & Paulson, Citation1992). However, it has been suggested that the formation of the β-sheet structure may be a prerequisite of aggregation and the formation of the cross-linked gel network, and parallel or anti-parallel β-sheet interacts with strong intermolecular hydrogen bonding at high temperatures, leading to the irreversible aggregation of protein and stabilization of the gel network (Wang & Damodaran, Citation1991). Where the percentage of secondary structure of the gel system does not change with temperature, we think that protein denaturation has ended, but it is possible that the content of some natural secondary structures may change (Sánchez-González et al., Citation2008). With increasing temperature, the α-helix of rabbit myosin gradually decreased, while the β-sheet tended to increase. This suggests that the loss of α-helix occurred simultaneously with the formation of β-sheet during the gelation process. The loss of α-helical structure probably led to the exposure of active groups such as sulfhydryl and hydrophobic groups, which resulted in chemical cross-linking induced by temperature, and formed a three-dimensional network retaining water.
4. Conclusion
This study was performed to provide a theoretical base for improved meat products, allowing the manipulation of processing conditions in order to obtain products with the desired structural and textural characteristics. CD analysis revealed that rabbit myosin possessed α-helix, β-sheet, and random coil structures. Gelation of myosin involved changes in myosin secondary conformation and myosin molecule–molecule and protein–water interactions. The gelation properties and secondary structures were strongly affected by temperature. The α-helix fraction declined with increasing temperature, while that of β-sheet and random coil content increased. The gel formed at temperatures of 42–45°C was tested using rheological methods, and these properties were consistent with the changes observed for WHC, hardness, and secondary structures. Heating caused the partial transformation of α-helix into β-sheet and random coil. Subsequently, myosin unfolded and aggregated, then formed a gel network.
Acknowledgments
This research was supported by Natural Science Foundation of Hebei Province (Grant No. C2013208014) and the National Natural Science Foundation (30771526), PR China. The assistance of Dr. Ron Tume (Food and Nutritional Sciences, CSIRO) is gratefully acknowledged.
References
- Andrade, M. A., Chacón, P., Merelo, J., & Morán, F. (1993). Evaluation of secondary structure of proteins from UV circular dichroism spectra using an unsupervised learning neural network. Protein Engineering Design and Selection, 6, 383–390. doi:10.1093/protein/6.4.383
- Bourne, M. (1978). Texture profile analysis. Food Technology, 32, 62–66, 72.
- Chan, J. K., Gill, T. A., & Paulson, A. T. (1992). The dynamics of thermal denaturation of fish myosins. Food Research International, 25, 117–123. doi:10.1016/0963-9969(92)90152-U
- Choi, S. M., & Ma, C. Y. (2007). Structural characterization of globulin from common buckwheat (Fagopyrum esculentum Moench) using circular dichroism and Raman spectroscopy. Food Chemistry, 102, 150–160. doi:10.1016/j.foodchem.2006.05.011
- Feng, Y., & Hultin, H. O. (2001). Effect of ph on the rheological and structural properties of gels of water-washed chicken-breast muscle at physiological ionic strength. Journal of Agricultural and Food Chemistry, 49, 3927–3935. doi:10.1021/jf001021f
- Fukushima, H., Satoh, Y., Yoon, S. H., Togashi, M., Nakaya, M., & Watabe, S. (2005). Rheological properties of fast skeletal myosin rod and light meromyosin from walleye pollack and white croaker: contribution of myosin fragments to thermal gel formation. Journal of Agricultural and Food Chemistry, 53, 9193–9198. doi:10.1021/jf051223h
- Greenfield, N. J. (1996). Methods to estimate the conformation of proteins and polypeptides from circular dichroism data. Analytical Biochemistry, 235, 1–10. doi:10.1006/abio.1996.0084
- Han, M. Y., Zhang, Y. J., Fei, Y., Xu, X. L., & Zhou, G. H. (2009). Effect of microbial transglutaminase on NMR relaxometry and microstructure of pork myofibrillar protein gel. European Food Research and Technology, 228, 665–670. doi:10.1007/s00217-008-0976-x
- Hermansson, A. M., & Buchheim, W. (1981). Characterization of protein gels by scanning and transmission electron microscopy A methodology study of soy protein gels. Journal of Colloid and Interface Science, 81, 519–530. doi:10.1016/0021-9797(81)90433-1
- Hermansson, A. M., Harbitz, O., & Langton, M. (1986). Formation of two types of gels from bovine myosin. Journal of the Science of Food and Agriculture, 37, 69–84. doi:10.1002/jsfa.2740370111
- Johnson, W. C. (1988). Secondary structure of proteins through circular dichroism spectroscopy. Annual Review of Biophysics and Biophysical Chemistry, 17, 145–166. doi:10.1146/annurev.bb.17.060188.001045
- Ker, Y. C., & Toledo, R. T. (1992). Influence of shear treatments on consistency and gelling properties of whey protein isolate suspensions. Journal of Food Science, 57, 82–85,90. doi:10.1111/j.1365-2621.1992.tb05430.x
- King, L., Seidel, J. C., & Lehrer, S. S. (1995). Unfolding domains in smooth muscle myosin rod. Biochemistry, 34, 6770–6774. doi:10.1021/bi00020a023
- Kocher, P. N., & Foegeding, E. A. (1993). Microcentrifuge-based method for measuring water-holding of protein gels. Journal of Food Science, 58, 1040–1046. doi:10.1111/j.1365-2621.1993.tb06107.x
- Li-Chan, E., & Nakai, S. (1991). Raman spectroscopic study of thermally and/or dithiothreitol induced gelation of lysozyme. Journal of Agricultural and Food Chemistry, 39, 1238–1245. doi:10.1021/jf00007a009
- Liu, R., Zhao, S. M., Xiong, S. B., Xie, B. J., & Qin, L. H. (2008). Role of secondary structures in the gelation of porcine myosin at different ph values. Meat Science, 80, 632–639. doi:10.1016/j.meatsci.2008.02.014
- Maltais, A., Remondetto, G. E., & Subirade, M. (2008). Mechanisms involved in the formation and structure of soya protein cold-set gels: A molecular and supramolecular investigation. Food Hydrocolloids, 22, 550–559. doi:10.1016/j.foodhyd.2007.01.026
- Samejima, K., Ishioroshi, M., & Yasui, T. (1981). Relative roles of the head and tail portions of the molecule in heat-induced gelation of myosin. Journal of Food Science, 46, 1412–1418. doi:10.1111/j.1365-2621.1981.tb04187.x
- Sánchez-González, I., Carmona, P., Moreno, P., Borderías, J., Sánchez-Alonso, I., Rodríguez-Casado, A., & Careche, M. (2008). Protein and water structural changes in fish Surimi during gelation as revealed by isotopic H/D exchange and Raman spectroscopy. Food Chemistry, 106, 56–64. doi:10.1016/j.foodchem.2007.05.067
- Sano, T., Noguchi, S. F., Matsumoto, J. J., & Tsuchiya, T. (1990). Thermal gelation characteristics of myosin subfragments. Journal of Food Science, 55, 55–58. doi:10.1111/j.1365-2621.1990.tb06015.x
- Wang, C., & Damodaran, S. (1991). Thermal gelation of globular proteins: influence of protein conformation on gel strength. Journal of Agricultural and Food Chemistry, 39, 433–438. doi:10.1021/jf00003a001
- Wang, S. F., & Smith, D. M. (1994). Heat-induced denaturation and rheological properties of chicken breast myosin and f-actin in the presence and absence of pyrophosphate. Journal of Agricultural and Food Chemistry, 42, 2665–2670. doi:10.1021/jf00048a003
- Westphalen, A. D., Briggs, J. L., & Lonergan, S. M. (2005). Influence of pH on rheological properties of porcine myofibrillar protein during heat induced gelation. Meat Science, 70, 293–299. doi:10.1016/j.meatsci.2005.01.015
- Westphalen, A. D., Briggs, J. L., & Lonergan, S. M. (2006). Influence of muscle type on rheological properties of porcine myofibrillar protein during heat-induced gelation. Meat Science, 72, 697–703. doi:10.1016/j.meatsci.2005.09.021
- Whitmore, L., & Wallace, B. (2004). DICHROWEB, an online server for protein secondary structure analyses from circular dichroism spectroscopic data. Nucleic Acids Research, 32, W668–W673. doi:10.1093/nar/gkh371
- Xiong, Y. L. (1997). Structure-function relationships of muscle protein. In S. Damodaran & A. Paraf (Eds.), Food protein and their application (pp. 341–391). New York: Marcel Dekker, Inc.
- Xiong, Y. L., & Blanchard, S. P. (1994). Myofibrillar protein gelation: viscoelastic changes related to heating procedures. Journal of Food Science, 59, 734–738. doi:10.1111/j.1365-2621.1994.tb08115.x
- Xiong, Y. L., & Brekke, C. J. (1990). Thermal transitions of salt-soluble proteins from pre- and postrigor chicken muscles. Journal of Food Science, 55, 1540–1543. doi:10.1111/j.1365-2621.1990.tb03563.x
- Xu, X. L., Han, M. Y., Fei, Y., & Zhou, G. H. (2011). Raman spectroscopic study of heat-induced gelation of pork myofibrillar proteins and its relationship with textural characteristic. Meat Science, 87, 159–164. doi:10.1016/j.meatsci.2010.10.001
- Yamamoto, K. (1990). Electron microscopy of thermal aggregation of myosin. Journal of Biochemistry, 108, 896–898.