ABSTRACT
African swine fever (ASF) is a devastating disease with a high impact on the pork industry worldwide. ASF virus (ASFV) is a very complex pathogen, the sole member of the family Asfaviridae, which induces a state of immune suppression in the host through infection of myeloid cells and apoptosis of lymphocytes. Moreover, haemorrhages are the other main pathogenic effect of ASFV infection in pigs, related to the infection of endothelial cells, as well as the activation and structural changes of this cell population by proinflammatory cytokine upregulation within bystander monocytes and macrophages. There are still many gaps in the knowledge of the role of proteins produced by the ASFV, which is related to the difficulty in producing a safe and effective vaccine to combat the disease, although few candidates have been approved for use in Southeast Asia in the past couple of years.
Introduction
African swine fever (ASF) is a highly contagious viral disease of swine, with a mortality rate that can reach to 100%, leading to devastating effects on domestic pig (Sus scrofa) populations and the pork industry worldwide [Citation1–4]. The disease is present in Africa, Europe, Asia, Oceania and the Americas (Caribbean) and, represents one of the major threats to the global farming industry. For these reasons, ASF is one of the diseases listed by the World Organization for Animal Health [Citation5]. The clinical presentation and pathology of ASF in domestic pigs may vary depending on the virulence of the virus isolate, individual features of the host, and route and dose of infection [Citation1,Citation6]. Regarding the virulence of the aetiological agent, ASF virus (ASFV) isolates are typically classified as highly virulent, moderately virulent, or low virulent [Citation7,Citation8] and their clinical presentation ranges from acute haemorrhagic fever and death to mild clinical signs and chronic infection [Citation1,Citation6,Citation9–11].
Apart from domestic pigs, Eurasian wild boar and feral pigs (also Sus scrofa) are highly susceptible to infection, which contribute to its spreading [Citation12–19]; however wild African suids such as warthogs (Phacochoerus africanus), bushpigs (Potamochoerus larvatus) and red river hogs (Potamochoerus porcus) can be infected by ASFV without showing clinical signs and acting as natural reservoir hosts [Citation1,Citation20–26]. Asian wild swine species are also susceptible to ASFV and some populations, such as the bearded pig (Sus barbatus, Sus ahoenobarbus) in Malaysia and Indonesia are being decimated by ASFV infection [Citation27]. Other wild pigs species in Southeast Asia species such as the pygmy hog (Porcula salvinia), warty pigs (Sus celebencis, Sus verrucosus, Sus cebiforns; Sus philippensis, Sus oliveri) and babirusas (Babyrousa spp.) are also in danger [Citation27], creating a large problem in food security and endangering the ecosystems [Citation27,Citation28]. ASFV can also infect different species of soft ticks from the genus Ornithodoros (mainly O. erraticus and O. moubata complex members), serving as vectors and reservoirs of ASFV [Citation29–36].
Although transmission and persistence vary significantly between countries and environmental conditions, ASFV spreads easily through direct contact with infected animals and fomites, ingestion of contaminated pig products and tick bites [Citation37–42]. Thus, in the absence of other treatments, early detection, high biosecurity, and culling of infected animals seems to be essential for reducing the risk of virus transmission and preventing the total depopulation of the farm [Citation39,Citation40,Citation43–46]. A vaccine is and will always be the best strategy and method for controlling and preventing the current ASFV pandemic [Citation47–50]. However, except for Vietnam, where two vaccines have been recently approved for controlled use in the field [Citation51–54], no registered vaccines are available to combat the disease in the rest of the world [Citation48]. Experimental results have demonstrated that inactivated vaccines are not sufficiently immunogenic [Citation55–57] and could lead to further expansion of the disease [Citation58]. Moreover, live attenuated vaccines may induce persistent infection, low cross-protection between different strains, and challenging differentiation of infected from vaccinated animals [Citation48]. Insufficient biosafety measures and human behaviour have been identified as the mayor risks for ASF spread and distribution [Citation59,Citation60]. Thus, increasing biosafety measures in pig farms and surveillance programs, together with the control of wild swine populations is currently the best way to prevent the disease until an effective vaccine is developed for use worldwide [Citation48,Citation59].
Emergence and re-emergence of ASF
The first observation of the disease was made in the former British East Africa Protectorate (now Kenya) in the early 1900s by veterinary pathologist Robert Eustace Montgomery, who described the disease as “an observation of East African swine fever” [Citation20,Citation61]. Montgomery preferred to consider the disease as a virulent form of the known disease called now “Classical Swine Fever” or “Hog Cholera” [Citation61], even though early observations showed no cross-protection with classical swine fever antiserum and the clinic pathological presentation showed evident differences [Citation20,Citation62–65]. The disease was also observed and reported in other African territories by official veterinarians in the first half of the XXth century and was restricted to Africa [Citation66] until it jumped to Europe in 1957 due to contaminated waste containing infected pork products from Africa that were fed to local pigs in Portugal [Citation67]. Even though this first outbreak was controlled rapidly and successfully, ASF re-entered Portugal in similar circumstances in 1960. It spread rapidly throughout the Iberian Peninsula and produced outbreaks in a variety of European countries (Belgium, France, Italy, Malta, the Netherlands, and the Soviet Union) during the following years [Citation67–72]. In the 1970s, the disease also emerged through the import/export of contaminated pork products in Brazil, Cuba, Haiti and the Dominican Republic; all outbreaks in the Americas were successfully controlled [Citation73–77]. Except for the Italian island of Sardinia, where ASFV remained endemic [Citation78] together with Africa [Citation79], the disease was successfully eradicated in Europe at the end of the 20th century, thanks to strict control and eradication programs [Citation1,Citation10,Citation67,Citation77,Citation80].
ASF emerged in the Republic of Georgia in 2007, most likely through contaminated food via maritime transport, with high virulence [Citation81], affecting domestic pigs and wild boars, and rapidly spreading to neighbouring countries, including the Russian Federation, Armenia, Ukraine, and Azerbaijan [Citation30,Citation67,Citation82–86] and even countries with no pork industry, but with wild boar populations like Iran [Citation87]. The ASFV isolate producing this re-emergence was named “Georgia 2007/1” and was classified as highly virulent [Citation88].
Between 2014 and 2018, ASFV re-emerged in the European Union, with infections coming from eastern countries and starting in Lithuania, Poland, Latvia and Estonia [Citation6,Citation89–92] and later, in Hungary, the Czech Republic and Romania [Citation89,Citation93,Citation94]. In 2018, ASFV was detected in Asia for the first time, spreading very rapidly and affecting more than 16 countries on the continent, particularly China, with devastating consequences, halving the swine population due to the effect of the disease and the culling strategy to control it [Citation95,Citation96]. Vietnam was also one of the most affected countries, with the first outbreak in February 2019 in the northern part of the country near China [Citation97–102]. In Vietnam, ASFV outbreaks resulted in the death or culling of nearly 6 million of pigs, which was more than the 20% of the country’s pig population [Citation103]. In September of the same year, WOAH reported the presence of ASFV in Oceania, in Timor-Leste, followed by Papua New Guinea in 2020 [Citation100,Citation104]. In 2021, ASF re-emerged in the Caribbean with outbreaks being reported in the Dominican Republic and Haiti, where it continues to cause problems in domestic pig populations [Citation105–107]. A recent study developed by Sykes et al., in 2023, estimated that the introduction of ASF into the southeastern part of United States would result in 72 infected farms in the first two months of the outbreak, concluding as well that fast action could prevent up to 79% of virus spread in the region [Citation108].
In Europe, ASF has also spread further west in recent years [Citation109,Citation110]. After 40 years of absence of the disease, ASF was reported in January 2022 on the Italian mainland and continues to spread throughout the country with outbreaks in domestic pigs and wild boars [Citation111,Citation112]. In 2023, ASFV was confirmed for the first time on domestic pig farms in Bosnia and Herzegovina, Greece and Croatia [Citation113].
Outbreaks have been reported in all continents except Antarctica and even though the disease is controlled in some areas, it will continue to produce important economic losses until an effective vaccine linked to a “differentiating infected from vaccinated animals” (DIVA) test is developed [Citation49].
The African swine fever virus and its replication cycle
ASFV is a large, cytoplasmic, double stranded DNA virus belonging to the genus Asfivirus [Citation57,Citation114]. ASFV is the only member of the Asfaviridae family of viruses. Its genome ranges from 170 to 190 kbp that encodes 150–200 viral proteins, including 68 structural proteins and more than 100 non-structural proteins, depending on the viral isolate [Citation2,Citation59]. Electron microscopy studies performed by Carrascosa et al. in 1984 [Citation115] showed that ASFV virions are icosahedral with a diameter between 170–300 nm, formed by different concentric layers; the external envelope, icosahedral capsid, inner envelope, core shell, and central nucleoid [Citation57,Citation59,Citation115–119]. A schematic representation of the ASFV structure is shown in .
Figure 1. African swine fever virus structure and replication cycle. ASFV virions are organised in multilayer structure formed by the external or outer envelope, the icosahedral capsid, the inner envelope, the core shell, and the central nucleoid. Main proteins from each layer are also represented. The mechanism by which ASFV enters the host cells has been controversial, although potential receptors such as CD163, siglec1 (CD169), CD45 and CD203a have been suggested (1). Other mechanism of viral entry such as clathrin-mediated endocytosis, micropinocytosis and phagocytosis of hemadsorpted red blood cells have been proposed (2). Any of these processes will be followed by the early endosomal formation and viral uncoating (3). At the end of this step, naked cores are ready for viral replication (4). An early stage of intranuclear replication takes place in the nucleus and then another step of viral replication occurs in the cytoplasm, within the perinuclear zone, in the so-called viral factories (5a,b). After viral assembly and maturation (6), new virions will exit the cell through exocytosis budding transiently acquiring an envelope that is unstable and rapidly lost (7). Image created with BioRender.com.
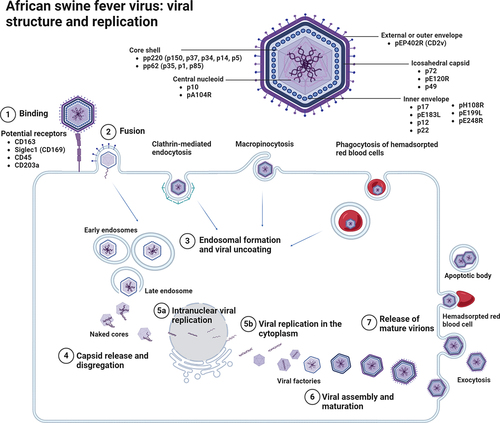
The external or outer envelope, composed of protein pEP402R, also named CD2v, is acquired from the host cellular membrane during budding [Citation59,Citation120]. The p12 protein is an important protein from the inner envelope involved in the attachment of virions to specific receptors on permissive cells and induces specific antibodies in naturally infected animals [Citation121–125]. The protein pEP402R, is involved in cell-cell adhesion, immune modulation, and virulence enhancement [Citation59,Citation118,Citation120]. It has been demonstrated that the pEP402R protein observed in the outer envelope of budding virions is responsible of the attachment to erythrocytes which would facilitate the spread of ASF infection in the host [Citation120]. Other proteins such as p24 are important components of the external envelope [Citation117,Citation120]. The capsid is composed of approximately 2800 capsomers with the appearance of hexagonal prisms [Citation117,Citation126]. p72 is the major capsid protein encoded by B646L gene, which has been historically used for genotyping of ASFV strains [Citation90,Citation127–129]. It constitutes approximately 30% of the total virion mass, and thus is one of the major proteins detected in AFSV infected animals, being highly antigenic and immunogenic [Citation117,Citation118,Citation130]. The pE120R is another important component of the viral capsid and is involved in the release of mature virions during viral replication [Citation117]. In the third layer, the inner envelope is a bilayer membrane derived from the endoplasmic reticulum and is formed by at least seven membrane viral proteins (p17, pE183L, p12, p22, pH108R, pE199L, and pE248R), with pE183R being the key protein involved in inner envelope formation [Citation120]. The core shell is formed by the proteolytic processing of two polyprotein precursors, pp220 and pp62, in many mature products to form the core shell. pp220 produces proteins p150, p37, p34, p14, and p5 whereas p35, p15, and p8 are derived from pp62 [Citation117,Citation120]. pp220 and p62 and their proteins are involved in the packaging and assembly of virions during ASFV infection [Citation118]. The nucleoid, a highly dense structure 80 nm in diameter, contains the viral genome and transcriptional machinery for the synthesis of early RNA and nucleoproteins for nucleoid assembly [Citation117]. Two main proteins, pA104R and p10, have been detected in the nucleoid which may play a role in the assembly of the DNA-containing nucleoid [Citation117,Citation120]. p10 and pA104R have been described as ideal targets for studying unexplored ASFV processes related to nucleoid formation and genome encapsulation [Citation120].
ASFV replicates mainly in monocyte-macrophage lineage cells, although other cell types can also be infected to a lesser extent [Citation131–134]. Moreover, it has been described that ASFV can also replicate in other cell types, including fibroblasts, endothelial cells, neutrophils, hepatocytes, and renal cells [Citation131,Citation133,Citation135,Citation136]. Infected cells usually appear swollen, showing pale intracytoplasmic inclusion bodies, visible under an optical microscope with semithin (1 µm) toluidine blue staining [Citation1]. The invasion of the host cell is determined by a sequence of successive steps, including virus binding, internalization, uncoating, early transcription-translation, genome replication, late protein synthesis, and virus particle morphogenesis, to finally release the viral progeny [Citation137]. Initial models of ASFV replication described a particular replication in the cytoplasm of the target cells, within the viral factories at the perinuclear level [Citation138], but recent research has postulated that ASFV also has an early stage of replication at the intranuclear level [Citation120,Citation139,Citation140]. The ASFV replication cycle is summarized in .
The mechanism by which ASFV enters host cells is controversial [Citation57,Citation141,Citation142]. In the early 1990s, Alcamí et al. [Citation119,Citation143,Citation144] described the presence of ASFV saturable receptors in the cellular plasma membrane of macrophages and Vero cells, however, subsequent studies showed that these receptors are necessary but not sufficient to guarantee the cell permissiveness of the virus [Citation145]. Early in vitro studies performed by Galindo et al. in 1997 [Citation121] demonstrated that the p12 protein is essential for viral attachment and that its specific interaction with membrane proteins on the surface of target cells is at least, one necessary step to produce infection. The scavenger receptor CD163, has been postulated to play a major role in the process of infection of porcine monocytes/macrophages by ASFV [Citation146], but CD163-knock-out pigs were still fully susceptible to ASFV challenge [Citation147], suggesting that other surface proteins could be also participate during the infection [Citation148], indicating the presence of other possible receptors such as CD45 or CD203a, which could be preferred for ASFV infection [Citation148]. Recently, Gao et al. in 2022 [Citation149] demonstrated in in vitro experiments that CD163 and Siglec1 (CD169) play a synergistic role in the process of AFSV infection. Nevertheless, further studies are needed to gain deeper insights into viral receptors during ASFV infection. Recently, it has been described that components of the MHC II system, particularly the membrane protein SLA-DM, is crucial for ASFV replication in vitro [Citation150].
Although its restricted tropism suggests a receptor-dependent mechanism of viral entry, such as dynamic-dependent and clathrin-mediated endocytosis [Citation137,Citation151], other mechanism of viral entry, such as micropinocytosis, which can be facilitated mechanistically by AXL, a tyrosine kinase receptor that interacts with ASFV externalised phosphatidyl serine on the envelope functioning as viral apoptotic mimicry [Citation152], and phagocytosis of hemadsorpted red blood cells have been described [Citation140,Citation153,Citation154]. Any of these processes increases the endosomal formation and migration in the intracellular space [Citation124]. CD1d can also facilitate the ASFV entry into the host cell via clathrin-mediated endocytosis [Citation155]. After the formation of the late endosome, the capsid disassembly, and the fusion of the ASFV inner envelope with the endosomal membrane occur [Citation141]. This involves disruption of the icosahedral capsid and dissociation of the external envelope, giving place to naked cores. It has been agreed on the fact that at this early stage of AFSV replication, viral DNA synthesis is primed in the nucleus and then full-length genomic DNA sequences are synthetized in the cytoplasm [Citation140]. Cytoplasmic replication occurs in the perinuclear zone, close to the microtubule organizing centre, in an area called viral replication sites or viral factories [Citation141,Citation156]. These viral factories are observed by transmission electron microscopy inside inclusion bodies [Citation1]. New virions are assembled in these viral factories and exit the cell through exocytosis budding, the formation of apoptotic bodies, or on the surface of hemadsorpted red blood cells [Citation117,Citation141].
Genetic diversity and virulence factors
ASFV is the sole arbovirus with a double stranded DNA (dsDNA) genome and is classified as the only member of the family Asfarviridae, within the proposed Megavirales order [Citation157,Citation158]. The first complete genome of ASFV was derived from the cell culture-adapted Badajoz71v (BA71v) strain [Citation159]. More recently, multiple sequences have been published and compared [Citation88,Citation160–162]. ASFV genome shows variable regions, and historically, ASFV isolates have been classified into 25 different genotypes based on partial sequencing of the B646L gene, which encodes the major capsid protein p72 [Citation163]. Genotype II has been responsible for most cases outside Africa and during the current pandemic, but also in many outbreaks in Africa.
Originally, the emergence of ASFV outside Africa was caused by genotype I ASFV; however, genotype II ASFV was responsible for the re-emergence of AFSV in the Caucasus region and its subsequent appearance in the rest of the European and Asian countries [Citation30,Citation81,Citation102,Citation114]. The outbreak of ASF in the Caucasus region was caused by a highly virulent genotype II, closely related to isolate Mkuzi 1979, which was isolated from a tick in Zululand near Mozambique [Citation88].
Genotypic diversity is highest in African countries [Citation164], whereas others with ASF outbreaks exhibit low genotype diversity (only genotypes I and II) [Citation112,Citation129]. In general, ASFV is very stable and shows a minimal mutation rate [Citation38]; however, recently, Zhao et al.. (2023) described three recombinants of genotype I and II in fields in China, which represents a new challenge for ASF control and vaccine development [Citation165]. These recombinants are genetically very similar and classified within genotype I according to the B646L gene sequencing; however, the 56% of their genomes are derived from genotype II [Citation165].
The use of p72 sequences has been invaluable in molecular epidemiology but it has also been erroneously applied to other purposes, such as prediction of cross-protection [Citation163]. The use of other genes, such as E183L, which encodes p54, has also been valuable for the epidemiological tracking of isolates [Citation102]. A novel proposed classification scheme for p72 reduces the number of genotypes from 25 to 6 [Citation166]. However, genotype classifications based on a single gene may not be ideal and the inclusion of multiple regions of the genomes considering the entire encoded proteome has been proposed to describe seven different biotypes using unsupervised machine learning [Citation163].
Genetic variation among ASFV genomes results mostly from the presence of different numbers of multi-gene family genes (MGF) in the left and right variable regions (LVR and RVR, respectively), with many functions still unknown [Citation167]. Different MGFs have been described and named according to their average number of encoded amino acids: MGF 100, 110, 300, 360 and 505/530 [Citation167]. Previous studies have suggested that MGF360, MGF505 and CD2v genes are important virulence factors in highly virulent genotype II strains isolated in Georgia in 2007 [Citation168]. Recently, Liu et al. identified I73R gene as an ASFV virulence-related gene [Citation169]. Deletion of this gene, which suppress the host innate immune response and promotes viral replication, has been proposed as a potential live attenuated vaccine candidate [Citation169]. MGF360-9 L is a major virulence factor that antagonize the JAK/STAT pathway [Citation170], while F317L inhibits NF-κB activation, what is associated with the ASFV strategy to evade the host immune response and promote viral replication [Citation171]. Deletion of a variety of genes in ASFV virulent isolates has been carried out to identify virulence factors and to test artificially attenuated viruses as potential vaccine candidates [Citation1,Citation52,Citation54,Citation136,Citation172–177]. The thymidine kinase (TK) gene has been shown to be involved in ASFV virulence, and its deletion resulted in reduced replication in primary macrophages culture and absence of clinical disease in experimentally infected pigs, but no protection against virus challenge with the parental ASFV isolate [Citation178]. I267L is another important virulence factor that inhibits polymerase III-RIG-I- mediated innate immunity [Citation179]. Deletion of H240R or E184L also partially reduces virulence [Citation180,Citation181]. Highly virulent strains with deletions in DP148R, DP71L, and DP96R have substantially attenuated the virulence in pigs [Citation182]. Multiple virulence factors are being studied in search of recombinant live attenuated vaccines as an attractive option to control ASF [Citation183].
Epidemiological cycles
Although the great importance of AFSV remains in its economic impact on domestic pig production, the natural hosts of ASFV are African wild suids, with wild boars and domestic pigs of all ages and breeds being also susceptible to the infection [Citation67]. Wild boar and feral pigs play a significant role in the epidemiology of ASF in Europe and Asia and are the main source of infection in recent outbreaks in Central Europe [Citation184–186]. Depending on the presence of wild reservoirs and ticks, and their interactions with domestic pigs, different epidemiological scenarios have been described: the sylvatic cycle, tick-pig cycle, and domestic cycle [Citation67]. A representation of the ASFV transmission cycle is shown in .
Figure 2. African swine fever transmission cycles. In Africa, the sylvatic cycle is maintained involving wildlife reservoirs including warthog (Phacochoerus africanus), bushpigs (Potamochoerus larvatus), red river hogs (Potamochoerus porcus) and giant forest hog (Hylochoerus meinertzhangeni) and the soft tick from genus Ornithodoros. The common warthog is very resistant to the ASFV infection, showing low viraemia and no evident clinical signs. Transmission between adult warthogs is infrequent but ASFV can persist long periods of time in tissues. Young warthogs can be infected by ASFV infected soft ticks, which become viraemic but, like adults, do not develop clinical disease. Bushpigs, red river hogs and giant forest hogs play a minor role in the maintenance of the sylvatic cycle in comparison with warthogs. Direct transmission between wild African suids and domestic pigs is unlikely. Soft ticks (O. moubata complex in Africa and O. erraticus in Europe) maintain the tick-pig cycle, being able to keep the infection during months or years and transmit it via sexual or transovarial to the progeny. The participation of the Eurasian wild boar (Sus scrofa) is crucial in the domestic cycle, which is maintained by the interaction between livestock and wildlife and poor biosecurity. Once ASFV is introduced into domestic pig populations, pigs transmit ASFV easily to other susceptible swine directly or indirectly. ASFV is also commonly transmitted indirectly through fomites or infected food. Image created with BioRender.com.
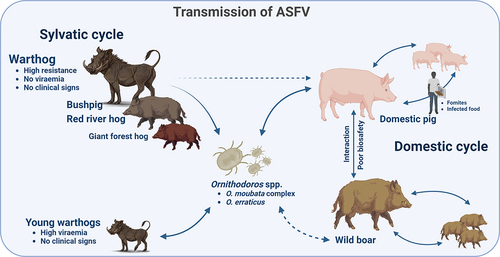
In Eastern and Southern Africa, ASF is maintained in an ancient sylvatic cycle involving wildlife reservoirs such as the common warthog (Phacochoerus africanus) and the soft ticks inhabiting their burrows [Citation187–189]. The common warthog is very resistant to ASFV infection, showing no evident clinical signs; however, infectious ASFV can persist in warthog tissues for long periods of time and perpetuate re-infections in soft ticks [Citation1,Citation190,Citation191]. Young warthogs can be infected by soft ticks carrying the virus, which become viraemic but do not develop clinical disease after ASFV infection [Citation191]. The virus can be found for 6–14 weeks in these animals [Citation192]. Naïve ticks are infected by feeding these viraemic juvenile warthogs [Citation30]. Although ASFV is present in adult warthog tissues, transmission between these animals is thought to be infrequent since viraemia is usually low [Citation30]. Direct transmission between warthogs and domestic pigs does not occur under experimental conditions, as the feeding of domestic pig with carcasses from hunted warthogs is an unlikely source of outbreaks in this species [Citation189].
Bushpigs (Potamochoerus larvatus) and red river hogs (Potamochoerus porcus), and other wild suids present across Central and Eastern Africa are also involved in this sylvatic cycle [Citation21,Citation24,Citation193]. Bushpigs show no clinical signs following ASFV infection in the same way than warthogs, but can also become viraemic and potentially infect domestic pigs [Citation23,Citation29,Citation194]. The viraemic period in these animals can last up to 34 weeks [Citation23]; however, their natural behaviours are less prone to interaction with soft tick vectors, and they exist in low numbers and are considered to play a minor role in the sylvatic cycle of AFSV in comparison with warthogs [Citation21,Citation189]. The giant forest hog (Hylochoerus meinertzhangeni) is a less common species of wild suids from Central Africa [Citation21,Citation195]. Only one giant forest hog infected with ASFV has been reported. Moreover, owing to its distribution, it is unlikely that this species has or will play a significant role in the transmission or dissemination of ASFV to domestic pigs [Citation21].
Maintenance of the tick-pig cycle involves the participation of Ornithodoros spp. ticks. The implication of arthropod vectors in the epidemiology of ASF has been speculated since the first description of the disease [Citation20]. However, during the 1960s, soft tick species from the genus Ornithodoros (O. erraticus) family Argasidae, were identified in Spain as vectors of ASFV that could maintain and transmit the disease [Citation196]. In Africa, O. moubata complex soft ticks are reservoirs of ASFV for both domestic and wild pigs [Citation114,Citation197–199]. Ornithodoros spp. ticks can maintain ASFV infection for months or even years after feeding viraemic animals and transmit it via sexual or transovarial route to the progeny [Citation30,Citation114]. Notably, AFSV is the only known DNA virus transmitted by arthropods [Citation30]. Forth and colleagues (2020) identified African Swine fever virus-like integrated (ASFLI) elements in the genome of O. moubata complex soft ticks by using transcriptome analysis and small RNA sequencing, corresponding to 10% of the ASFV genome suggesting that these arthropods are not only important for the transmission of the AFSV but also for the evolution [Citation200].
Another important epidemiological cycle of ASF without the involvement of soft tick affects wild boars and domestic pigs. The Eurasian wild boar (Sus scrofa) is a native wild species of most of Europe, Asia and Northern Africa, with an increasing population [Citation201], and has been introduced in other continents and islands [Citation202]. The high livestock-wildlife interaction in some European countries, together with poor biosafety measures, make this species a crucial participant within the domestic cycle. This has been occurring in the Russian Federation, trans-Caucasian countries, and Europe in recent years [Citation67,Citation203]. Once ASFV has been introduced into the domestic pig population, it transmits easily to other susceptible swine by direct contact [Citation30]. ASFV can also be transmitted through infected fomites, blood, body fluids, faeces and carcasses, or pork products from infected pigs, which are common indirect routes of transmission [Citation37,Citation204]. The illegal movement of live pigs or pork products also plays an important role in the appearance of new outbreaks on all continents [Citation205–207].
The ASF clinical and pathological features
ASFV infection in domestic pigs can follow different clinical courses, including peracute (or hyperacute), acute, subacute, and chronic [Citation1,Citation58]. The clinical presentation and associated gross and histopathological findings vary depending on the virulence of the virus strain, route and dose of infection, and immune status of the host [Citation1,Citation58]. One of the most important factors in the development of one or another clinical course and pathology is the virulence of the ASFV isolate/strain, which can be classified as highly virulent, moderately virulent or low virulent [Citation7].
The peracute form is typically induced by highly virulent strains and is characterized by a very rapid clinical course, with high temperatures (up to 42°C), anorexia, lethargy, and occasionally sudden death without signs of the disease [Citation1]. This clinical course is often observed when the virus enters a naïve pig population for the first time, for example, the first cases in one country before the explosion of acute clinical cases [Citation1,Citation102]. Some animals can show respiratory signs related to high fever, but no gross pathology or histopathological lesions are found on post-mortem examination [Citation1].
The acute clinical course is often observed in naïve farms after the first fatal cases have been reported because of infection with high or moderately virulent isolates. High temperatures (40–42ºC) were observed together with lethargy and anorexia. Affected animals tend to bunch up together, and many animals show centripetal cyanosis, which is easily observed in the ears, lower limbs, snout, tail, genitalia, and lower abdomen [Citation1]. Haemorrhages are often present in the skin, such as petechiae or ecchymosis, and nasal discharge stained with blood (epistaxis) is also a common finding [Citation1]. Respiratory signs are typically characterized by dyspnoea and pulmonary oedema is frequent in animals infected with highly virulent isolates [Citation208,Citation209]. Diarrhea and vomiting can be observed, and in particular dark stained faeces due to digested blood from gastrointestinal haemorrhages (melaena) and abortions may also occur in pregnant sows [Citation65,Citation210,Citation211]. At post-mortem examination, haemorrhages can be observed in several organs, being the haemorrhagic splenomegaly a characteristic lesion of this clinical course, showing a very dark spleen occupying a large space within the abdominal cavity [Citation212–214]. Multifocal haemorrhagic lymphadenitis is another typical lesion observed in acute ASF, mostly affecting the gastro-hepatic, renal, and mesenteric lymph nodes, although many other lymph nodes can be affected. Haemorrhages can also be found in other organs such as the kidney, intestine serosae and epicardium in the heart or urinary bladder [Citation1,Citation58,Citation135,Citation136,Citation213,Citation215,Citation216].
The subacute form can be observed in animals infected with rare moderately virulent isolates, showing similar but less marked clinical signs as in acute forms. Animals exhibit moderate to high temperatures with mortality ranging from 30 to 70%, compared to up to 100% in the acute form [Citation1,Citation58]. Moreover, animals die at days 7–20 after infection, in contrast to days 3–7 in the acute form [Citation1,Citation58]. Haemorrhagic changes are similar to those observed in the acute form, although they can present with higher intensity [Citation65]. On post-mortem examination, haemorrhagic lymphadenitis and splenomegaly were also observed, sometimes affecting only certain areas of the spleen [Citation1,Citation65]. Ascites, hydropericardium, and multifocal oedema, particularly in the perirenal fat and wall of the gall bladder, are common lesions observed [Citation65]. Petechial haemorrhages in the kidneys and other organs, and multifocal pneumonia can also be observed [Citation1].
The chronic clinical form of the disease is typically caused by infection with low virulence ASFV isolates. This clinical form is characterized by the presence of arthritis and chronic lesions in the skin, with secondary infections leading to necrosis, emaciation, growth retardation and abortion in pregnant sows [Citation196,Citation217]. Secondary bacterial infections are also observed in multiple organs, inducing pneumonia, tonsil necrosis, or fibrinous polyserositis [Citation217]. This clinical course was observed infrequently in the Iberian Peninsula and the Dominican Republic in the 1960s and the 1970s, and it has been associated with ASFV isolates that may have evolved from early failed vaccine trials in the Iberian Peninsula [Citation58]. The evolution from moderately and highly virulent isolates in other areas of the world where the virus has been present for long periods of time has not produced this chronic form of the disease [Citation58,Citation218], although an attenuation of the virulence has been observed with increased frequency in some countries and these “naturally attenuated” isolates are being evaluated as potential vaccine candidates to protect from heterologous highly virulent infection [Citation11,Citation219–221]. These animals infected with attenuated ASFV strains present an epidemiological challenge as infected animals can become carriers of the virus for long periods of time [Citation220,Citation222].
Pathogenesis of ASFV infection and immune response
The main histopathological lesions observed in typical acute cases of ASF are haemorrhages and lymphoid depletion [Citation1,Citation65,Citation136,Citation214,Citation223–227], similar to many haemorrhagic fevers affecting a variety of mammalian species [Citation228–231]. Severe leucopenia, mostly associated with lymphopenia and a general state of immunodeficiency, is characteristic of ASF [Citation226,Citation232].
The pathogenesis of ASFV is summarized in . Animals get infected by the oral-nasal route from another infected animal or after the bite of an infected soft tick [Citation1]. ASFV replicates initially in the tonsil or regional lymph nodes [Citation233,Citation234], spreading to other organs via the lymph or blood [Citation235–237]. The main target cells for ASFV are monocytes and macrophages; hence, lymphoid tissues are important in the pathogenesis of the disease and as diagnostic material [Citation131,Citation208,Citation214]. At the early stages of infection, AFSV inhibits apoptosis in infected cells, allowing viral replication and spreading [Citation2]. However, at later stages of infection, viral replication induces apoptosis and necrosis, not only in infected cells, but also in bystander cells [Citation225,Citation226]. Virions are released in the blood, lymph, or the interstitial space [Citation225,Citation238–240].
Figure 3. Pathogenesis of African swine fever infection in pigs. Animals get infected by oral-nasal route from another infected animal or through infected soft ticks (Ornothodoros erraticus and O. moubata complex) which act as vectors. Initial replication takes place in lymphoid tissues such as spleen, lymph nodes, tonsils or thymus in the case of young animals. The main target cells for ASFV are macrophages and monocytes, while other cell types can also be infected to a lesser extent. At early stage of infection, AFSV induces an inhibition of the apoptosis in infected cells to allow viral replication and spreading. After replication in the monocyte-macrophage cell population, ASFV induces the secretion of proinflammatory cytokines IL-1, IL-6, IL-8 and TNF-α. This called “cytokine storm” causes an induction of necrotic and apoptotic pathways not only in infected cells but also in bystander cells such as lymphocytes and endothelial cells. This massive destruction of immune cells is related with the severe immunosuppression observed in ASFV infected animals. The cytokine storm is also responsible of the endothelial dysfunction which takes place after ASFV infection and will induces multiorgan oedema and haemorrhages. The pathogenic mechanism of early haemorrhages is related to the phagocytic activation of capillary endothelial cells, that induce cell hypertrophy and exposure of the capillary basal membrane, prompting the activation of the coagulation cascade and inducing disseminated intravascular coagulation (D.I.C.). Late haemorrhages, observed in different organs, such as spleen, lymph nodes or kidneys are related with a loss of intercellular junctions of the blood vessels and the exposure of the basal lamina, initiating the coagulation cascade, platelet aggregation and fibrin deposition. Image created with BioRender.com.
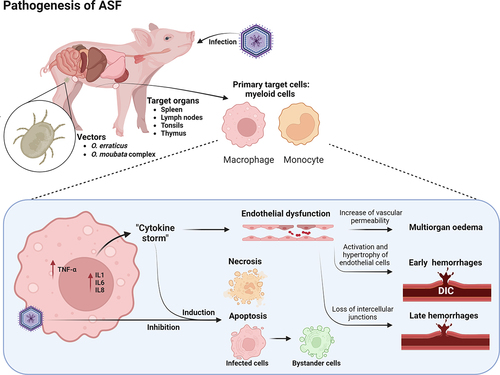
During the acute phase of ASF, massive destruction of the lymphatic system is observed [Citation1,Citation223,Citation226,Citation227] and a large proportion of macrophages and T and B cells undergo cell death, predominantly lymphocyte apoptosis [Citation226,Citation227,Citation241]. The replication of ASFV in the monocyte-macrophage cell population induces profound changes in the cell, first activating the secretion of proinflammatory cytokines, such as interleukin-1 (IL-1), TNF-α, IL-6 or IL-8 [Citation214,Citation223,Citation226,Citation242–244]. This so-called “cytokine storm,” observed in many other viral diseases affecting animals and humans [Citation245,Citation246] is responsible for the severe induction of apoptosis in lymphocytes in the neighbouring cells surrounding infected monocytes and macrophages [Citation226,Citation227]. This massive destruction of immune cells is related to the significant immunosuppression observed in infected animals. However, there are some differences in the regulation of this pro-inflammatory response between the low- and high-virulence strains. Low virulent ASFV isolates may induce higher levels of these cytokines in vitro in comparison with highly virulent strains, suggesting that these highly virulent strains can modulate the inflammatory response to evade the host immune response [Citation247].
The other major pathogenic effect of ASFV infection is haemorrhage. ASF can be considered a haemorrhagic fever with many similar pathogenic mechanisms observed in human haemorrhagic fevers, such as filovirus infections [Citation228–230]. The most typical macroscopic lesion of acute ASFV is haemorrhagic/hyperaemic splenomegaly, with distinct severity depending on the virulence of the ASFV strain involved [Citation1,Citation210]. At the microscopic level, the spleen shows a hyperaemic red pulp with abundant red blood cells, platelet thrombi, and cell debris, disrupting the normal architecture of the organ [Citation132,Citation226,Citation248]. Infected splenic macrophages fixed to smooth muscle fibres, a distinct cell population of the porcine spleen [Citation248], induce the loss of intercellular junctions and the exposure of the basal lamina, initiating the coagulation cascade, platelet aggregation, and fibrin deposition [Citation224,Citation249]. Haemorrhages are also frequent in other organs without the macrophages population fixed to smooth muscle cells, such as the lymph nodes, kidneys, or skin [Citation224]. These haemorrhages can be observed at the early stages of the disease, although infection of endothelial cells only occurs at later time points [Citation132,Citation250]. The mechanism of early haemorrhage is related to the phagocytic activation of capillary endothelial cells, which induce cell hypertrophy and exposure of the capillary basal membrane prompting the activation of the coagulation cascade and inducing disseminated intravascular coagulation (D.I.C.) [Citation224,Citation237,Citation251]. Structural changes in megakaryocytes are also observed and contribute to the state of thrombocytopenia during subacute ASF, with abundant denuded megakaryocytes in the bone marrow, a feature also observed in other haemorrhagic fevers such as Classical Swine fever [Citation224,Citation237,Citation251–253].
Multiorgan oedema has also been observed during most clinical courses of ASF [Citation1]. In the lungs, infection of intravascular macrophages (PIMs) induces secretory activation in infected and neighbouring uninfected cells [Citation254]. These enlarged activated cells produce large quantities of proinflammatory cytokines, including IL-1α and TNF-α [Citation255]. These cytokines possess chemotactic activity and increase the vascular permeability, leading to fluid leakage into the alveolar and interalveolar spaces [Citation255]. Hypo-oncotic oedema is also observed in many organs owing to the low protein levels in the blood because of the marked anorexia in infected animals. Moreover, hepatic congestion is also a typical feature of ASF with histopathological changes, including multifocal periportal infiltrates, infection of Kupffer cells, and hepatocytes at later stages of infection [Citation133,Citation134,Citation136,Citation256,Citation257].
These differences in pathogenesis and clinical outcomes are the result of different immune responses to ASFV. Immune responses against ASFV are generally quite complex and poorly understood, making it difficult to generatr of vaccines to combat the disease [Citation140,Citation258]. The main cell targets of ASFV are within the myeloid lineage, but T cell responses are heavily affected by infection [Citation225,Citation226,Citation258]. There are many gaps in the knowledge of the role of unconventional T cells, especially gamma-delta T cells, which can have a potential role in the induction of further responses against infection [Citation259,Citation260].
Diagnostic techniques and differential diagnosis
Owing to the lethal consequences of ASFV infection in domestic pigs, reliable and fast diagnostic methods are of high importance. Clinicopathological diagnosis is very important when the disease is spreading to neighbouring countries or farms at a local level. The farm veterinarian and the official veterinary service should be aware of the situation and the typical features of the disease to be ready to perform a confirmatory laboratory diagnosis. Some diseases can induce similar clinical signs and lesions, even similar mortality as in the case of classical swine fever, which has been the most important differential diagnosis for ASF for many decades [Citation261–265]. The recent emergence of highly pathogenic Porcine Reproductive and Respiratory Syndrome (PRRS) in Asia and Eastern Europe has also added this disease to the potential clinicopathological differential diagnosis with ASF, as infected animals can display high morbidity and mortality, haemorrhage and lymphoid depletion [Citation266–268].
The laboratory diagnosis of ASF has improved radically in the past few years, alongside the rapid expansion of the disease. The hemadsorption [Citation269] test has been a basic ASFV diagnostic technique for many decades [Citation270,Citation271]. It is considered a definitive diagnostic test for ASFV detection, but it requires specialized laboratories, primary cell preparation, and takes more than a week to obtain the results, which are normally used in reference laboratories [Citation272]. Moreover, some ASFV isolates are non-hemadsorbing due to deletion of the EP402R gene, which encodes for the CD2v protein responsible for hemadsorption [Citation161]. For these reasons, molecular tests for the detection of ASFV nucleic acid, with high sensitivity and specificity, are the most employed worldwide and are recommended by the WOAH [Citation273–275]. Generally, these molecular methods target a highly conserved region of the ASFV genome that encodes for the capsid protein p72 (B646L gene) [Citation272]. Real-time PCR is widely considered the gold standard for the detection of ASFV nucleic acid in diagnostic samples, but isothermal amplification techniques have gained popularity owing to the swift method and the lack of thermal amplification [Citation276,Citation277]. New isothermal amplification methods including loop-mediated (LAMP), rolling circle (RCA), and recombinase polymerase (RPA) have been recently described and used for ASFV detection [Citation278–282]. CRISPR-associated proteins, engineered via gene-editing techniques are promising next-generation tools for the diagnosis of infectious diseases, including ASF [Citation161,Citation272,Citation283].
The detection of antibodies against ASFV is peripheral blood has been another crucial approach for the detection and monitoring of ASF, and WOAH recommends certain antibody immunoassays. Among these diagnostic techniques, ELISAs has been widely used [Citation284,Citation285]. Lateral flow devices used in the field have also been developed recently showing good sensitivity and specificity [Citation286]. However, the majority of animals suffering acute clinical course, the typical one observed during the current pandemic, die before seroconversion occurs, making antibody tests less useful in many situations.
Control measures and vaccines against ASF
Prevention is the most important tool to prevent ASF from entering an ASF-free zone or country. Monitoring domestic and wild pig populations is a key measure for preventing ASF, and many countries have implemented robust surveillance plans. There are many potential routes for the introduction of ASFV into a new territory, including legal and illegal movements of animals and pork products, which can travel long distances, or through infected wild boar [Citation287–289]. Once a disease is present in a country, a combination of control tools is used to eradicate the disease. These include robust diagnostics, improved biosecurity, elimination of infected animals, and the restriction of animal/fomite movement. These measures have been used successfully to eradicate ASF in Europe and incursions in America in the past century. However, ASF has become a pandemic and control and eradication measures have only limited success [Citation49]. Research on effective and safe vaccines has become crucial for ASF control [Citation290].
Vaccination strategies should not be used as a stand-alone intervention to control ASF and should be conducted under well-established programs together with post-vaccination surveillance monitoring of the disease and biosecurity [Citation291].
Inactivated, subunit, mRNA, DNA, or viral vectored vaccine candidates have not provided effective and reliable protection, with only a few reports showing some protection against challenge [Citation292–296], and only one showing 100% protection after challenge with a moderately virulent isolate, using a pool of eight different ASFV antigens [Citation297].
The attenuated vaccine strategy is more feasible, showing good protection when pigs are immunized and survive after challenge with virulent strains [Citation49,Citation298]. However, there are concerns regarding potential virulence reversion, and in-depth safety studies are necessary before deploying a vaccine in the field [Citation299]. As live-attenuated vaccines can replicate successfully within the host, both humoral and cellular responses are triggered and adjuvants with co-stimulatory activity are not required to enhance the quality and magnitude of the immune response [Citation47].
Live attenuated vaccines have shown potential protection from homologous ASFV challenge, but not for heterologous isolates, from the first experiments in the 1960s [Citation49]. However, safety concerns were also raised with these preliminary vaccination attempts, as some animals would not be protected and mortality and/or clinical signs and lesions were observed [Citation49,Citation300,Citation301]
Attenuation of ASFV through deletion of various genes has been widely reported [Citation52–54,Citation136,Citation176,Citation180,Citation181,Citation183,Citation302]. A highly effective attenuated vaccine has been developed by deleting the I177L gene, achieving sterile immunity at medium or higher doses and protecting pigs from the virulent strains circulating in Eurasia [Citation52]. This vaccine has been licenced for use in Vietnam together with another vaccine with a deletion in MGF. Naturally attenuated ASFV isolates have also shown potential as vaccine candidates, showing partial and/or full protection against homologous or heterologous ASFV challenge [Citation221,Citation303–306].
Genotype I ASFV emerged in China in 2021, three years after the first report of a Georgia07-like genotype II strain, and started causing chronic infections, low virulence, and effective transmissibility, with many similarities to the attenuated NH/P68 and OURT88/3 isolates from Portugal [Citation11]. Genotype I and II AFSV recombinants were identified in the field in China in 2021, which presents a new challenge to vaccine development, as some recombinant attenuated vaccines based on genotype II have shown good protection against genotype II isolates but not against genotype I/II recombinant virulent strains [Citation165]
The use of non-compliant and poor-quality vaccines poses a major risk to the pig industry [Citation291]. The emergence of chronic clinical courses in the Iberian Peninsula in the 20th century has been associated with the use of failed vaccination strategies [Citation67]. Additionally, these vaccines could recombine with field isolates creating novel strains that could evade the current diagnostic techniques and result in persistent infections in farms [Citation291].
Conclusions and future considerations
Pork products account for more than a third of global meat consumption; hence, ASF continues to be a major threat not only to the pork industry but also to food security. It has been rapidly expanded worldwide. The infectious agent ASFV is complex, containing many determinants of virulence and strategies to escape the host immune response. There are still knowledge gaps regarding the role of proteins produced by ASFV and in the immune response after infection. More research is also needed on the correlates of protection.
For the first time, vaccines against ASF have been approved for use in Southeast Asia, and more vaccine candidates are in the pipeline. A safe and efficacious vaccine coupled with a specific high-throughput DIVA diagnostic technique, integrated into a robust control and eradication program to understand the different socio-economic and epidemiological scenarios, would provide the basis for the eradication of the disease.
Acknowledgements
The authors would like to acknowledge many colleagues from the ASF scientific community, including the University of Córdoba and Centro de Investigacion en Sanidad Animal (CISA-INIA) in Spain, the Vietnam National University of Agriculture (Hanoi, Vietnam), and The Pirbright Institute (United Kingdom).
Disclosure statement
No potential conflict of interest was reported by the author(s).
Data availability statement
Data sharing is not applicable to this article as no new data were created or analysed in this study.
Additional information
Funding
References
- Salguero FJ. Comparative pathology and pathogenesis of African swine fever infection in Swine. Front Vet Sci. 2020;7:282. doi: 10.3389/fvets.2020.00282. Epub 2020/06/09. PubMed PMID: 32509811; PubMed Central PMCID: PMC7248413.
- Dixon LK, Sun H, Roberts H. African swine fever. Antiviral Res. 2019;165:34–25. doi: 10.1016/j.antiviral.2019.02.018. Epub 2019/03/06. PubMed PMID: 30836106.
- Ward MP, Tian K, Nowotny N. African swine fever, the forgotten pandemic. Transbound Emerg Dis. 2021;68(5):2637–2639. doi: 10.1111/tbed.14245. Epub 2021/09/10. PubMed PMID: 34499823.
- Penrith ML. African swine fever: transboundary diseases. Onderstepoort J Vet Res. 2009;76(1):91–95. doi:10.4102/ojvr.v76i1.70. Epub 2009/12/09. PubMed PMID: 19967933
- Anonymous. WOAH listed diseases. 2024. https://www.woah.org/en/what-we-do/animal-health-and-welfare/animal-diseases/
- Sánchez-Vizcaíno JM, Mur L, Gomez-Villamandos JC, et al. An update on the epidemiology and pathology of African swine fever. J Comp Pathol. 2015;152(1):9–21. doi: 10.1016/j.jcpa.2014.09.003. Epub 2014/12/03. PubMed PMID: 25443146.
- Pan IC, Hess WR. Virulence in African swine fever: its measurement and implications. Am J Vet Res. 1984;45(2):361–366. Epub 1984/02/01. PubMed PMID: 6711963.
- Forth JH, Calvelage S, Fischer M, et al. African swine fever virus – variants on the rise. Emerg Microbes Infect. 2023;12(1):2146537. doi: 10.1080/22221751.2022.2146537. Epub 2022/11/11. PubMed PMID: 36356059; PubMed Central PMCID: PMC9793911.
- Zhu JJ, Ramanathan P, Bishop EA, et al. Mechanisms of African swine fever virus pathogenesis and immune evasion inferred from gene expression changes in infected swine macrophages. PLOS ONE. 2019;14(11):e0223955. doi: 10.1371/journal.pone.0223955. Epub 2019/11/15. PubMed PMID: 31725732.
- Blome S, Franzke K, Beer M. African swine fever – a review of current knowledge. Virus Res. 2020;287:198099. doi: 10.1016/j.virusres.2020.198099. Epub 2020/08/07. PubMed PMID: 32755631.
- Sun E, Huang L, Zhang X, et al. Genotype I African swine fever viruses emerged in domestic pigs in China and caused chronic infection. Emerg Microbes Infect. 2021;10(1):2183–2193. doi: 10.1080/22221751.2021.1999779. Epub 2021/10/29. PubMed PMID: 34709128; PubMed Central PMCID: PMC8635679.
- Sánchez-Cordón PJ, Nunez A, Neimanis A, et al. African swine fever: disease dynamics in wild boar experimentally infected with ASFV isolates belonging to genotype I and II. Viruses. 2019;11(9):852. doi: 10.3390/v11090852. Epub 2019/09/22. PubMed PMID: 31540341; PubMed Central PMCID: PMC6783972.
- Pikalo J, Zani L, Hühr J, et al. Pathogenesis of African swine fever in domestic pigs and European wild boar–lessons learned from recent animal trials. Virus Res. 2019;271:197614. doi: 10.1016/j.virusres.2019.04.001. Epub 2019/04/07. PubMed PMID: 30953662.
- Lentz HHK, Bergmann H, Conraths FJ, et al. The diffusion metrics of African swine fever in wild boar. Sci Rep. 2023;13(1):15110. doi: 10.1038/s41598-023-42300-0. Epub 2023/09/14. PubMed PMID: 37704714; PubMed Central PMCID: PMC10499946.
- Jo YS, Gortázar C. African swine fever in wild boar: assessing interventions in South Korea. Transbound Emerg Dis. 2021;68(5):2878–2889. doi: 10.1111/tbed.14106. Epub 2021/04/13. PubMed PMID: 33844467.
- Koh EY, Tan AKS, Yeo D, et al. Detection of African swine fever virus from wild boar, Singapore, 2023. Emerg Infect Dis. 2023;29(12):2580–2583. doi: 10.3201/eid2912.230966. Epub 2023/09/15. PubMed PMID: 37708842; PubMed Central PMCID: PMC10683832.
- Schulz K, Masiulis M, Staubach C, et al. African swine fever and its epidemiological course in Lithuanian wild boar. Viruses. 2021;13(7):1276. doi: 10.3390/v13071276. Epub 2021/07/03. PubMed PMID: 34208894; PubMed Central PMCID: PMC8310040.
- Rogoll L, Güttner AK, Schulz K, et al. Seasonal occurrence of African swine fever in wild boar and domestic pigs in EU member states. Viruses. 2023;15(9):1955. doi: 10.3390/v15091955. Epub 2023/09/28. PubMed PMID: 37766361; PubMed Central PMCID: PMC10536336.
- Fekede RJ, HaoNing W, Hein VG, et al. Could wild boar be the trans-Siberian transmitter of African swine fever? Transbound Emerg Dis. 2021;68(3):1465–1475. doi: 10.1111/tbed.13814. Epub 2020/09/01. PubMed PMID: 32866334.
- Montgomery RE. On a form of swine fever occurring in British East Africa (Kenya colony). J Comp Pathol And Ther. 1921;34:159–191. doi: 10.1016/S0368-1742(21)80031-4.
- Jori F, Bastos AD. Role of wild suids in the epidemiology of African swine fever. Ecohealth. 2009;6(2):296–310. doi: 10.1007/s10393-009-0248-7. Epub 2009/11/17. PubMed PMID: 19915917.
- Oberin M, Hillman A, Ward MP, et al. The potential role of wild suids in African swine fever spread in Asia and the pacific region. Viruses. 2022;15(1):61. doi: 10.3390/v15010061. Epub 2023/01/22. PubMed PMID: 36680101; PubMed Central PMCID: PMC9867030.
- Anderson EC, Hutchings GH, Mukarati N, et al. African swine fever virus infection of the bushpig (potamochoerus porcus) and its significance in the epidemiology of the disease. Vet Microbiol. 1998;62(1):1–15. doi: 10.1016/s0378-1135(98)00187-4. Epub 1998/07/11. PubMed PMID: 9659687.
- Oura CA, Powell PP, Anderson E, et al. The pathogenesis of African swine fever in the resistant bushpig. J Gen Virol. 1998;79(6):1439–1443. doi: 10.1099/0022-1317-79-6-1439. Epub 1998/06/20. PubMed PMID: 9634086.
- Penrith ML, Bastos AD, Etter EMC, et al. Epidemiology of African swine fever in Africa today: sylvatic cycle versus socio-economic imperatives. Transbound Emerg Dis. 2019;66(2):672–686. doi: 10.1111/tbed.13117. Epub 2019/01/12. PubMed PMID: 30633851.
- Jori F, Vial L, Penrith ML, et al. Review of the sylvatic cycle of African swine fever in sub-Saharan Africa and the Indian ocean. Virus Res. 2013;173(1):212–227. doi: 10.1016/j.virusres.2012.10.005. Epub 2012/11/13. PubMed PMID: 23142551.
- Luskin MS, Meijaard E, Surya S, et al. African swine fever threatens Southeast Asia’s 11 endemic wild pig species. 2021;14(3):e12784. doi: 10.1111/conl.12784.
- Meijaard E, Erman A, Ancrenaz M, et al. Pig virus imperils food security in borneo. Science. 2024;383(6680):267. doi: 10.1126/science.adn3857.
- Roger F, Ratovonjato J, Vola P, et al. Ornithodoros porcinus ticks, bushpigs, and African swine fever in madagascar. Exp Appl Acarol. 2001;25(3):263–269. doi: 10.1023/a:1010687502145. Epub 2001/08/29. PubMed PMID: 11523921.
- Gaudreault NN, Madden DW, Wilson WC, et al. African swine fever virus: an emerging DNA arbovirus. Front Vet Sci. 2020;7:215. doi: 10.3389/fvets.2020.00215. Epub 2020/06/02. PubMed PMID: 32478103; PubMed Central PMCID: PMC7237725.
- Jori F, Bastos A, Boinas F, et al. An updated review of ornithodoros ticks as reservoirs of African swine fever in sub-Saharan Africa and Madagascar. Pathogens. 2023;12(3):469. doi: 10.3390/pathogens12030469. Epub 2023/03/30. PubMed PMID: 36986391; PubMed Central PMCID: PMC10059854.
- Kleiboeker SB, Scoles GA. Pathogenesis of African swine fever virus in ornithodoros ticks. Anim Health Res Rev. 2001;2(2):121–128. doi: 10.1079/AHRR200133. Epub 2002/02/08. PubMed PMID: 11831434.
- Anholt H, Hillman V, Vaughan J, et al. The soft tick ornithodoros moubata and its role in the epidemiology of African swine fever in Central Malawi. J Wildl Dis. 2023;59(3):465–471. doi: 10.7589/jwd-d-22-00090. Epub 2023/05/12. PubMed PMID: 37170355.
- Basto AP, Nix RJ, Boinas F, et al. Kinetics of African swine fever virus infection in ornithodoros erraticus ticks. J Gen Virol. 2006;87(Pt 7):1863–1871. doi: 10.1099/vir.0.81765-0. Epub 2006/06/09. PubMed PMID: 16760388.
- Pereira de Oliveira R, Hutet E, Paboeuf F, et al. Comparative vector competence of the Afrotropical soft tick ornithodoros moubata and palearctic species, O. erraticus and O. verrucosus, for African swine fever virus strains circulating in Eurasia. PLOS ONE. 2019;14(11):e0225657. doi: 10.1371/journal.pone.0225657. Epub 2019/11/28. PubMed PMID: 31774871; PubMed Central PMCID: PMC6881060.
- Kleiboeker SB, Burrage TG, Scoles GA, et al. African swine fever virus infection in the argasid host, ornithodoros porcinus porcinus. J Virol. 1998;72(3):1711–1724. doi: 10.1128/jvi.72.3.1711-1724.1998. Epub 1998/03/14. PubMed PMID: 9499019; PubMed Central PMCID: PMC109458.
- Guinat C, Gubbins S, Vergne T, et al. Experimental pig-to-pig transmission dynamics for African swine fever virus, Georgia 2007/1 strain. Epidemiol Infect. 2016;144(1):25–34. doi: 10.1017/S0950268815000862. Epub 2015/05/21. PubMed PMID: 25989921; PubMed Central PMCID: PMC4697298.
- Dixon LK, Stahl K, Jori F, et al. African swine fever epidemiology and control. Annu. Rev. Anim. Biosci. 2020;8(1):221–246. doi: 10.1146/annurev-animal-021419-083741. Epub 2019/11/20. PubMed PMID: 31743062.
- Juszkiewicz M, Walczak M, Woźniakowski G, et al. African swine fever: transmission, spread, and control through biosecurity and disinfection, including polish trends. Viruses. 2023;15(11):2275. doi: 10.3390/v15112275. Epub 2023/11/25. PubMed PMID: 38005951; PubMed Central PMCID: PMC10674562.
- Liu Y, Zhang X, Qi W, et al. Prevention and control strategies of African swine fever and progress on pig farm repopulation in China. Viruses. 2021;13(12):2552. doi: 10.3390/v13122552. Epub 2021/12/29. PubMed PMID: 34960821; PubMed Central PMCID: PMC8704102.
- Main AR, Halasa T, Olesen AS, et al. Estimating transmission dynamics of African swine fever virus from experimental studies. Transbound Emerg Dis. 2022;69(6):3858–3867. doi: 10.1111/tbed.14757. Epub 2022/11/09. PubMed PMID: 36346271; PubMed Central PMCID: PMC10098825.
- Guinat C, Gogin A, Blome S, et al. Transmission routes of African swine fever virus to domestic pigs: current knowledge and future research directions. Vet Rec. 2016;178(11):262–267. doi: 10.1136/vr.103593. Epub 2016/03/12. PubMed PMID: 26966305; PubMed Central PMCID: PMC4819659.
- Mutua F, Dione M. The context of application of biosecurity for control of African swine fever in smallholder pig systems: current gaps and recommendations. Front Vet Sci. 2021;8:689811. doi: 10.3389/fvets.2021.689811. Epub 2021/08/20. PubMed PMID: 34409087; PubMed Central PMCID: PMC8364973.
- Klein L, Hessling-Zeinen S, Adler F, et al. Exploring pig farmers‘ decision-making concerning biosecurity measures against African swine fever. Prev Vet Med. 2023;217:105949. doi: 10.1016/j.prevetmed.2023.105949. Epub 2023/06/08. PubMed PMID: 37285701.
- Ekakoro JE, Nawatti M, Singler DF, et al. A survey of biosecurity practices of pig farmers in selected districts affected by African swine fever in Uganda. Front Vet Sci. 2023;10:1245754. doi: 10.3389/fvets.2023.1245754. Epub 2023/09/04. PubMed PMID: 37662985; PubMed Central PMCID: PMC10469975.
- Turlewicz-Podbielska H, Kuriga A, Niemyjski R, et al. African swine fever virus as a difficult opponent in the fight for a vaccine—current data. Viruses. 2021;13(7):1212. doi: 10.3390/v13071212. Epub 2021/07/03. PubMed PMID: 34201761; PubMed Central PMCID: PMC8310326.
- Urbano AC, Ferreira F. African swine fever control and prevention: an update on vaccine development. Emerg Microbes Infect. 2022;11(1):2021–2033. doi: 10.1080/22221751.2022.2108342. Epub 2022/08/02. PubMed PMID: 35912875; PubMed Central PMCID: PMC9423837.
- Zhang H, Zhao S, Zhang H, et al. Vaccines for African swine fever: an update. Front Microbiol. 2023;14:1139494. doi: 10.3389/fmicb.2023.1139494. Epub 2023/05/14. PubMed PMID: 37180260; PubMed Central PMCID: PMC10173882.
- Muñoz-Pérez C, Jurado C, Sánchez-Vizcaíno JM. African swine fever vaccine: turning a dream into reality. Transbound Emerg Dis. 2021;68(5):2657–2668. doi: 10.1111/tbed.14191. Epub 2021/06/18. PubMed PMID: 34137198.
- Rock DL. Challenges for African swine fever vaccine development-“ … perhaps the end of the beginning”. Vet Microbiol. 2017;206:52–58. doi: 10.1016/j.vetmic.2016.10.003. Epub 2016/10/21. PubMed PMID: 27756505.
- Tran XH, Le TTP, Nguyen QH, et al. African swine fever virus vaccine candidate ASFV-G-ΔI1s77L efficiently protects European and native pig breeds against circulating Vietnamese field strain. Transbound Emerg Dis. 2022;69(4):e497–e504. doi: 10.1111/tbed.14329. Epub 2021/09/29. PubMed PMID: 34582622.
- Borca MV, Ramirez-Medina E, Silva E, et al. Development of a highly effective African swine fever virus vaccine by deletion of the I177L gene results in sterile immunity against the current epidemic Eurasia strain. J Virol. 2020;94(7). doi: 10.1128/jvi.02017-19. Epub 2020/01/24. PubMed PMID: 31969432; PubMed Central PMCID: PMC7081903.
- Borca MV, Rai A, Espinoza N, et al. African swine fever vaccine candidate ASFV-G-ΔI177L produced in the swine macrophage-derived cell line IPKM remains genetically stable and protective against homologous virulent challenge. Viruses. 2023;15(10):2064. doi: 10.3390/v15102064. Epub 2023/10/28. PubMed PMID: 37896841; PubMed Central PMCID: PMC10612016.
- Gladue DP, Ramirez-Medina E, Vuono E, et al. Deletion of the A137R gene from the pandemic strain of African swine fever virus attenuates the strain and offers protection against the virulent pandemic virus. J Virol. 2021;95(21):e0113921. doi: 10.1128/jvi.01139-21. Epub 2021/08/19. PubMed PMID: 34406865; PubMed Central PMCID: PMC8513468.
- Cadenas-Fernández E, Sánchez-Vizcaíno JM, van den Born E, et al. High doses of inactivated African swine fever virus are safe, but do not confer protection against a virulent challenge. Vaccines (Basel). 2021;9(3):242. doi: 10.3390/vaccines9030242. Epub 2021/04/04. PubMed PMID: 33802021; PubMed Central PMCID: PMC7999564.
- Goatley LC, Reis AL, Portugal R, et al. A pool of eight virally vectored African swine fever antigens protect pigs against fatal disease. Vaccines (Basel). 2020;8(2):234. doi: 10.3390/vaccines8020234. Epub 2020/05/24. PubMed PMID: 32443536; PubMed Central PMCID: PMC7349991.
- Revilla Y, Pérez-Núñez D, Richt JA. African swine fever virus biology and vaccine approaches. Adv Virus Res. 2018;100:41–74. doi: 10.1016/bs.aivir.2017.10.002. Epub 2018/03/20. PubMed PMID: 29551143.
- Sanchez-Vizcaino JM, Mur L, Gomez-Villamandos JC, et al. An update on the epidemiology and pathology of African swine fever. J Comp Pathol. 2015;152(1):9–21. doi: 10.1016/j.jcpa.2014.09.003. Epub 2014/12/03. PubMed PMID: 25443146.
- Wang F, Zhang H, Hou L, et al. Advance of African swine fever virus in recent years. Res Vet Sci. 2021;136:535–539. doi: 10.1016/j.rvsc.2021.04.004. Epub 2021/04/22. PubMed PMID: 33882382.
- Bellini S, Casadei G, De Lorenzi G, et al. A review of risk factors of African swine fever incursion in pig farming within the European Union scenario. Pathogens. 2021;10(1):84. doi: 10.3390/pathogens10010084. Epub 2021/01/23. PubMed PMID: 33478169; PubMed Central PMCID: PMC7835761.
- Penrith ML, Kivaria FM. One hundred years of African swine fever in Africa: where have we been, where are we now, where are we going? Transbound Emerg Dis. 2022;69(5):e1179–e200. doi: 10.1111/tbed.14466. Epub 2022/02/02. PubMed PMID: 35104041.
- Maurer FD, Griesemer RA. The pathology of African swine fever; a comparison with hog cholera. Am J Vet Res. 1958;19(72):517–539. Epub 1958/07/01. PubMed PMID: 13559600.
- Salmon DE. The virus of hog cholera. Public health papers and reports. Public Health Pap Rep. 1885;11:73–77. Epub 1885/01/01. PubMed PMID: 19600258; PubMed Central PMCID: PMC2266199.
- Blome S, Staubach C, Henke J, et al. Classical swine fever—an updated review. Viruses. 2017;9(4):86. doi: 10.3390/v9040086. Epub 2017/04/22. PubMed PMID: 28430168; PubMed Central PMCID: PMC5408692.
- Gomez-Villamandos JC, Carrasco L, Bautista MJ, et al. African swine fever and classical swine fever: a review of the pathogenesis. Dtsch Tierarztl Wochenschr. 2003;110(4):165–169. Epub 2003/05/22. PubMed PMID: 12756959.
- Hammond RA, Detray DE. A recent case of African swine fever in Kenya, East Africa. J Am Vet Med Assoc. 1955;126(938):389–391. Epub 1955/05/01. PubMed PMID: 14367196.
- Sanchez-Vizcaino JM, Mur L, Martinez-Lopez B. African swine fever: an epidemiological update. Transbound Emerg Dis. 2012;59(Suppl 1):27–35. doi: 10.1111/j.1865-1682.2011.01293.x. Epub 2012/01/10. PubMed PMID: 22225967.
- Biront P, Castryck F, Leunen J. An epizootic of African swine fever in Belgium and its eradication. Vet Rec. 1987;120(18):432–434. doi: 10.1136/vr.120.18.432. Epub 1987/05/02. PubMed PMID: 3603981.
- Swaney LM, Lyburt F, Mebus CA, et al. Genome analysis of African swine fever virus isolated in Italy in 1983. Vet Microbiol. 1987;14(2):101–104. doi: 10.1016/0378-1135(87)90001-0. Epub 1987/06/01. PubMed PMID: 2821672.
- Terpstra C, Wensvoort G. African swine fever in the Netherlands. Tijdschr Diergeneeskd. 1986;111(8):389–392. Epub 1986/04/15. PubMed PMID: 3518145.
- Wilkinson PJ, Wardley RC, Williams SM. African swine fever virus (Malta/78) in pigs. J Comput Pathol. 1981;91(2):277–284. doi: 10.1016/0021-9975(81)90033-5. Epub 1981/04/01. PubMed PMID: 7345110.
- Korennoy FI, Gulenkin VM, Gogin AE, et al. Estimating the basic reproductive number for African swine fever using the Ukrainian historical epidemic of 1977. Transbound Emerg Dis. 2017;64(6):1858–1866. doi: 10.1111/tbed.12583. Epub 2016/09/27. PubMed PMID: 27667658.
- [Preliminary report on the African swine fever epizootic in Cuba. Methods of diagnosis and control]. Bull Off Int Epizoot. 1971;75(7):367–437. Epub 1971/07/01. PubMed PMID: 5168939.
- Alexander FC. Experiences with African swine fever in Haiti. Ann NY Acad Sci. 1992;653(1):251–256. doi: 10.1111/j.1749-6632.1992.tb19654.x. Epub 1992/06/16. PubMed PMID: 1626878.
- Mebus CA, Dardiri AH, Hamdy FM, et al. Some characteristics of African swine fever viruses isolated from Brazil and the Dominican Republic. Proc Annu Meet U S Anim Health Assoc. 1978;(82):232–236. Epub 1978/01/01. PubMed PMID: 287093.
- Reichard RE. African swine fever in the Americas. Proc Annu Meet US Anim Health Assoc. 2024;1978(82):226–231. Epub 1978/01/01. PubMed PMID: 287092.
- DE Paula Lyra LTM, Saraiva VEV, Hermida Lage GR, et al. Eradication of African swine fever from Brazil. Rev Sci Tech. 1986;5(3):771–787. doi: 10.20506/rst.5.3.261. Epub 1986/09/01. PubMed PMID: 32736448.
- Laddomada A, Rolesu S, Loi F, et al. Surveillance and control of African swine fever in free-ranging pigs in Sardinia. Transbound Emerg Dis. 2019;66(3):1114–1119. doi: 10.1111/tbed.13138. Epub 2019/02/05. PubMed PMID: 30715791; PubMed Central PMCID: PMC6849606.
- Penrith ML, Vosloo W. Review of African swine fever: transmission, spread and control. J S Afr Vet Assoc. 2009;80(2):58–62. doi: 10.4102/jsava.v80i2.172. Epub 2009/10/17. PubMed PMID: 19831264.
- Costard S, Wieland B, de Glanville W, et al. African swine fever: how can global spread be prevented? Philos Trans R Soc Lond B Biol Sci. 2009;364(1530):2683–2696. doi: 10.1098/rstb.2009.0098. Epub 2009/08/19. PubMed PMID: 19687038; PubMed Central PMCID: PMC2865084.
- Rowlands RJ, Michaud V, Heath L, et al. African swine fever virus isolate, Georgia, 2007. Emerg Infect Dis. 2008;14(12):1870–1874. doi: 10.3201/eid1412.080591. Epub 2008/12/03. PubMed PMID: 19046509; PubMed Central PMCID: PMC2634662.
- Oganesyan AS, Petrova ON, Korennoy FI, et al. African swine fever in the Russian federation: spatio-temporal analysis and epidemiological overview. Virus Res. 2013;173(1):204–211. doi: 10.1016/j.virusres.2012.12.009. Epub 2013/01/01. PubMed PMID: 23274108.
- Sánchez-Vizcaíno JM, Mur L, Martínez-López B. African swine fever (ASF): five years around Europe. Vet Microbiol. 2013;165(1–2):45–50. doi: 10.1016/j.vetmic.2012.11.030. Epub 2012/12/26. PubMed PMID: 23265248.
- Sargsyan MA, Voskanyan HE, Karalova EM. Third wave of African swine fever infection in Armenia: Virus demonstrates the reduction of pathogenicity. Vet World. 2018;11(1):5–9. doi: 10.14202/vetworld.2018.5-9. Epub 2018/02/27. PubMed PMID: 29479149; PubMed Central PMCID: PMC5813512.
- Bezymennyi M, Tarasov O, Kyivska GV, et al. Epidemiological characterization of African swine fever dynamics in Ukraine, 2012–2023. Vaccines (Basel). 2023;11(7):1145. doi: 10.3390/vaccines11071145. Epub 2023/07/29. PubMed PMID: 37514961; PubMed Central PMCID: PMC10384127.
- Kolbasov D, Titov I, Tsybanov S, et al. African swine fever virus, Siberia, Russia, 2017. Emerg Infect Dis. 2018;24(4):796–798. doi: 10.3201/eid2404.171238. Epub 2018/03/20. PubMed PMID: 29553323; PubMed Central PMCID: PMC5875268.
- Rahimi P, Sohrabi A, Ashrafihelan J, et al. Emergence of African swine fever virus, northwestern Iran. Emerg Infect Dis. 2010;16(12):1946–1948. doi: 10.3201/eid1612.100378. Epub 2010/12/03. PubMed PMID: 21122227; PubMed Central PMCID: PMC3294588.
- Chapman David AG, Darby AC, Da Silva M, et al. Genomic analysis of highly virulent Georgia 2007/1 isolate of African swine fever virus. Emerg Infect Dis. 2011;17(4):599–605. doi: 10.3201/eid1704.101283. Epub 2011/04/08. PubMed PMID: 21470447; PubMed Central PMCID: PMC3379899.
- Cwynar P, Stojkov J, Wlazlak K. African swine fever status in Europe. Viruses. 2019;11(4):310. doi: 10.3390/v11040310. Epub 2019/04/03. PubMed PMID: 30935026; PubMed Central PMCID: PMC6521326.
- Gallardo C, Fernandez-Pinero J, Pelayo V, et al. Genetic variation among African swine fever genotype II viruses, eastern and central Europe. Emerg Infect Dis. 2014;20(9):1544–1547. doi: 10.3201/eid2009.140554. Epub 2014/08/26. PubMed PMID: 25148518; PubMed Central PMCID: PMC4178389.
- Olsevskis E, Guberti V, Serzants M, et al. African swine fever virus introduction into the EU in 2014: experience of Latvia. Res Vet Sci. 2016;105:28–30. doi: 10.1016/j.rvsc.2016.01.006. Epub 2016/04/02. PubMed PMID: 27033903.
- Nurmoja I, Motus K, Kristian M, et al. Epidemiological analysis of the 2015-2017 African swine fever outbreaks in Estonia. Prev Vet Med. 2020;181. doi: 10.1016/j.prevetmed.2018.10.001. Epub 2018/11/30. PubMed PMID: 30482617.
- Ungur A, Cazan CD, Panait LC, et al. Genotyping of African swine fever virus (ASFV) isolates in Romania with the first report of genotype II in symptomatic pigs. Vet Sci. 2021;8(12):290. doi: 10.3390/vetsci8120290. Epub 2021/12/24. PubMed PMID: 34941817; PubMed Central PMCID: PMC8706303.
- Martínez-Avilés M, Iglesias I, De La Torre A. Evolution of the ASF infection stage in wild boar within the EU (2014-2018). Front Vet Sci. 2020;7:155. doi: 10.3389/fvets.2020.00155. Epub 2020/04/17. PubMed PMID: 32296720; PubMed Central PMCID: PMC7141172.
- Zhou L, Yu EYW, Wang S, et al. African swine fever epidemic in China. Veterinary Record. 2019;184(23):713. doi: 10.1136/vr.l4026. Epub 2019/06/09. PubMed PMID: 31175249.
- Li X, Tian K. African swine fever in China. Vet Rec. 2018;183(9):300–301. doi: 10.1136/vr.k3774. Epub 2018/09/09. PubMed PMID: 30194128.
- Le VP, Jeong DG, Yoon SW, et al. Outbreak of African swine fever, Vietnam, 2019. Emerg Infect Dis. 2019;25(7):1433–1435. doi: 10.3201/eid2507.190303. Epub 2019/05/11. PubMed PMID: 31075078; PubMed Central PMCID: PMC6590755.
- Shao Q, Li R, Han Y, et al. Temporal and spatial evolution of the African swine fever epidemic in Vietnam. Int J Environ Res Public Health. 2022;19(13):8001. doi: 10.3390/ijerph19138001. Epub 2022/07/10. PubMed PMID: 35805660; PubMed Central PMCID: PMC9265385.
- Mighell E, Ward MP. African swine fever spread across Asia, 2018-2019. Transbound Emerg Dis. 2021;68(5):2722–2732. doi: 10.1111/tbed.14039. Epub 2021/02/19. PubMed PMID: 33599077.
- Tran HTT, Truong AD, Dang AK, et al. Genetic characterization of African swine fever viruses circulating in North Central region of Vietnam. Transbound Emerg Dis. 2021;68(3):1697–1699. doi: 10.1111/tbed.13835. Epub 2020/09/18. PubMed PMID: 32939964.
- Nga BTT, Padungtod P, Depner K, et al. Implications of partial culling on African swine fever control effectiveness in Vietnam. Front Vet Sci. 2022;9:957918. doi: 10.3389/fvets.2022.957918. Epub 2022/09/20. PubMed PMID: 36118335; PubMed Central PMCID: PMC9479321.
- Nga BTT, Tran Anh Dao B, Nguyen Thi L, et al. Clinical and pathological study of the first outbreak cases of African swine fever in Vietnam, 2019. Front Vet Sci. 2020;7:392. doi: 10.3389/fvets.2020.00392. Epub 2020/08/01. PubMed PMID: 32733925; PubMed Central PMCID: PMC7360720.
- Nguyen-Thi T, Pham-Thi-Ngoc L, Nguyen-Ngoc Q, et al. An assessment of the economic impacts of the 2019 African swine fever outbreaks in Vietnam. Front Vet Sci. 2021;8:686038. doi: 10.3389/fvets.2021.686038. Epub 2021/11/12. PubMed PMID: 34760953; PubMed Central PMCID: PMC8573105.
- Barnes TS, Morais O, Cargill C, et al. First steps in managing the challenge of African swine fever in Timor-Leste. One Health. 2020;10:100151. doi: 10.1016/j.onehlt.2020.100151. Epub 2020/10/30. PubMed PMID: 33117869; PubMed Central PMCID: PMC7582221.
- Gonzales W, Moreno C, Duran U, et al. African swine fever in the Dominican Republic. Transbound Emerg Dis. 2021;68(6):3018–3019. doi: 10.1111/tbed.14341. Epub 2021/10/06. PubMed PMID: 34609795.
- Jean-Pierre RP, Hagerman AD, Rich KM. An analysis of African swine fever consequences on rural economies and smallholder swine producers in Haiti. Front Vet Sci. 2022;9:960344. doi: 10.3389/fvets.2022.960344. Epub 2022/11/01. PubMed PMID: 36311651; PubMed Central PMCID: PMC9597192.
- Ruiz-Saenz J, Diaz A, Bonilla-Aldana DK, et al. African swine fever virus: a re-emerging threat to the swine industry and food security in the Americas. Front Microbiol. 2022;13:1011891. doi: 10.3389/fmicb.2022.1011891. Epub 2022/10/25. PubMed PMID: 36274746; PubMed Central PMCID: PMC9581234.
- Sykes AL, Galvis JA, O’Hara KC, et al. Estimating the effectiveness of control actions on African swine fever transmission in commercial swine populations in the United States. Preventive Veterinary Medicine. 2023;217:105962. doi: 10.1016/j.prevetmed.2023.105962. Epub 2023/06/25. PubMed PMID: 37354739.
- Sauter-Louis C, Schulz K, Richter M, et al. African swine fever: Why the situation in Germany is not comparable to that in the Czech Republic or Belgium. Transbound Emerg Dis. 2022;69(4):2201–2208. doi: 10.1111/tbed.14231. Epub 2021/07/12. PubMed PMID: 34247453.
- Richter M, Schulz K, Elflein T, et al. The first eighteen months of African swine fever in wild boar in Saxony, Germany and Latvia—a comparison. Pathogens. 2023;12(1):87. doi: 10.3390/pathogens12010087. Epub 2023/01/22.PubMed PMID: 36678435; PubMed Central PMCID: PMC9867452.
- Pavone S, Iscaro C, Dettori A. African swine fever: the state of the art in Italy. Animals (Basel). 2023;13(19):2998. doi: 10.3390/ani13192998. Epub 2023/10/14. PubMed PMID: 37835604; PubMed Central PMCID: PMC10571570.
- Giammarioli M, Alessandro D, Cammà C, et al. Molecular characterization of the first African swine fever virus genotype II strains identified from Mainland Italy, 2022. Pathogens. 2023;12(3):372. doi: 10.3390/pathogens12030372. Epub 2023/03/30. PubMed PMID: 36986294; PubMed Central PMCID: PMC10055901.
- Brellou GD, Tassis PD, Apostolopoulou EP, et al. Report on the first African swine fever case in Greece. Vet Sci. 2021;8(8):163. doi: 10.3390/vetsci8080163. Epub 2021/08/27. PubMed PMID: 34437485; PubMed Central PMCID: PMC8402752.
- Costard S, Mur L, Lubroth J, et al. Epidemiology of African swine fever virus. Virus Res. 2013;173(1):191–197. doi: 10.1016/j.virusres.2012.10.030. Epub 2012/11/06. PubMed PMID: 23123296.
- Carrascosa JL, Carazo JM, Carrascosa AL, et al. General morphology and capsid fine structure of African swine fever virus particles. Virology. 1984;132(1):160–172. doi: 10.1016/0042-6822(84)90100-4. Epub 1984/01/15. PubMed PMID: 6695498.
- Andres G, Simon-Mateo C, Vinuela E. Assembly of African swine fever virus: role of polyprotein. J Virol. 1997;71(3):2331–2341. doi: 10.1128/jvi.71.3.2331-2341.1997. Epub 1997/03/01. PubMed PMID: 9032369; PubMed Central PMCID: PMC191342.
- Salas ML, Andres G. African swine fever virus morphogenesis. Virus Res. 2013;173(1):29–41. doi: 10.1016/j.virusres.2012.09.016. Epub 2012/10/13. PubMed PMID: 23059353.
- Jia N, Ou Y, Pejsak Z, et al. Roles of African swine fever virus structural proteins in viral infection. J Vet Res. 2017;61(2):135–143. doi: 10.1515/jvetres-2017-0017. Epub 2018/07/07. PubMed PMID: 29978065; PubMed Central PMCID: PMC5894393.
- Alcami A, Carrascosa AL, Vinuela E. The entry of African swine fever virus into vero cells. Virology. 1989;171(1):68–75. doi: 10.1016/0042-6822(89)90511-4. Epub 1989/07/01. PubMed PMID: 2741349.
- Alejo A, Matamoros T, Guerra M, et al. A proteomic atlas of the African swine fever virus particle. J Virol. 2018;92(23). doi: 10.1128/JVI.01293-18. Epub 2018/09/07. PubMed PMID: 30185597; PubMed Central PMCID: PMC6232493.
- Galindo I, Vinuela E, Carrascosa AL. Protein cell receptors mediate the saturable interaction of African swine fever virus attachment protein p12 with the surface of permissive cells. Virus Res. 1997;49(2):193–204. doi: 10.1016/s0168-1702(97)00037-3. Epub 1997/06/01. PubMed PMID: 9213394.
- Alcami A, Angulo A, Lopez-Otin C, et al. Amino acid sequence and structural properties of protein p12, an African swine fever virus attachment protein. J Virol. 1992;66(6):3860–3868. doi: 10.1128/jvi.66.6.3860-3868.1992. Epub 1992/06/01. PubMed PMID: 1583732; PubMed Central PMCID: PMC241171.
- Angulo A, Vinuela E, Alcami A. Inhibition of African swine fever virus binding and infectivity by purified recombinant virus attachment protein p12. J Virol. 1993;67(9):5463–5471. doi: 10.1128/jvi.67.9.5463-5471.1993. Epub 1993/09/01. PubMed PMID: 8350406; PubMed Central PMCID: PMC237948
- Duan X, Ru Y, Yang W, et al. Research progress on the proteins involved in African swine fever virus infection and replication. Front Immunol. 2022;13:947180. doi: 10.3389/fimmu.2022.947180. Epub 2022/08/09. PubMed PMID: 35935977; PubMed Central PMCID: PMC9353306.
- Zhang K, Li S, Liu S, et al. Spatiotemporally orchestrated interactions between viral and cellular proteins involved in the entry of African swine fever virus. Viruses. 2021;13(12):2495. doi: 10.3390/v13122495. Epub 20211213. PubMed PMID: 34960765; PubMed Central PMCID: PMC8703583.
- Wang G, Xie M, Wu W, et al. Structures and functional diversities of ASFV proteins. Viruses. 2021;13(11):2124. doi: 10.3390/v13112124. Epub 20211021. PubMed PMID: 34834930; PubMed Central PMCID: PMC8619059.
- Bastos AD, Penrith ML, Cruciere C, et al. Genotyping field strains of African swine fever virus by partial p72 gene characterisation. Arch Virol. 2003;148(4):693–706. doi: 10.1007/s00705-002-0946-8. Epub 2003/03/29. PubMed PMID: 12664294.
- Gallardo C. African swine fever virus p72 genotype IX in domestic pigs, Congo, 2009. Emerg Infect Dis. 2011;17(8):1556–1558. doi: 10.3201/eid1708.101877. Epub 2011/08/02. PubMed PMID: 21801650; PubMed Central PMCID: PMC3381578.
- Gallardo C, Casado N, Soler A, et al. A multi gene-approach genotyping method identifies 24 genetic clusters within the genotype II-European African swine fever viruses circulating from 2007 to 2022. Front Vet Sci. 2023;10:1112850. doi: 10.3389/fvets.2023.1112850. Epub 2023/02/11. PubMed PMID: 36761884; PubMed Central PMCID: PMC9905734.
- Liu L, Atim S, LeBlanc N, et al. Overcoming the challenges of pen-side molecular diagnosis of African swine fever to support outbreak investigations under field conditions. Transbound Emerg Dis. 2019;66(2):908–914. doi: 10.1111/tbed.13103. Epub 2018/12/17. PubMed PMID: 30554469.
- Carrasco L, de Lara FC, Martin de las Mulas J, et al. Virus association with lymphocytes in acute African swine fever. Vet Res. 1996;27(3):305–312. Epub 1996/01/01. PubMed PMID: 8767892.
- Carrasco L, Chacon F, Martin de Las Mulas J, et al. Ultrastructural changes related to the lymph node haemorrhages in acute African swine fever. Res Vet Sci. 1997;62(3):199–204. doi: 10.1016/s0034-5288(97)90190-9. Epub 1997/05/01. PubMed PMID: 9300534.
- Gomez-Villamandos JC, Hervas J, Mendez A, et al. A pathological study of the perisinusoidal unit of the liver in acute African swine fever. Res Vet Sci. 1995;59(2):146–151. doi: 10.1016/0034-5288(95)90049-7. Epub 1995/09/01. PubMed PMID: 8525104.
- Sierra MA, Bernabe A, Mozos E, et al. Ultrastructure of the liver in pigs with experimental African swine fever. Vet Pathol. 1987;24(5):460–462. doi: 10.1177/030098588702400516. Epub 1987/09/01. PubMed PMID: 3672813.
- Gomez-Villamandos JC, Hervas J, Mendez A, et al. Pathological changes in the renal interstitial capillaries of pigs inoculated with two different strains of African swine fever virus. J Comput Pathol. 1995;112(3):283–298. doi: 10.1016/s0021-9975(05)80081-7. Epub 1995/04/01. PubMed PMID: 7560303.
- Salguero FJ, Gil S, Revilla Y, et al. Cytokine mRNA expression and pathological findings in pigs inoculated with African swine fever virus (E-70) deleted on A238L. Vet Immunol Immunopathol. 2008;124(1–2):107–119. doi: 10.1016/j.vetimm.2008.02.012. Epub 2008/04/04. PubMed PMID: 18384883.
- Galindo I, Cuesta-Geijo MA, Hlavova K, et al. African swine fever virus infects macrophages, the natural host cells, via clathrin- and cholesterol-dependent endocytosis. Virus Res. 2015;200:45–55. doi: 10.1016/j.virusres.2015.01.022. Epub 2015/02/11. PubMed PMID: 25662020.
- Chapman DA, Netherton CL, Dixon LK. African swine fever virus replication and genomics. Virus Res. 2013;173(1):3–14. doi: 10.1016/j.virusres.2012.10.020. Epub 2012/11/13. PubMed PMID: 23142553.
- Simoes M, Martins C, Ferreira F. Early intranuclear replication of African swine fever virus genome modifies the landscape of the host cell nucleus. Virus Res. 2015;210:1–7. doi: 10.1016/j.virusres.2015.07.006. Epub 2015/07/18. PubMed PMID: 26183880.
- Netherton CL, Shimmon GL, Hui JYK, et al. African Swine Fever Virus Host–Pathogen Interactions. In: Vijayakrishnan S, Y Jiu J Harris, editors. Virus Infected Cells. Cham: Springer International Publishing; 2023. p. 283–331.
- Galindo I, Alonso C. African swine fever virus: a review. Viruses. 2017;9(5):103. doi: 10.3390/v9050103. Epub 2017/05/11. PubMed PMID: 28489063; PubMed Central PMCID: PMC5454416.
- Sanchez EG, Perez-Nunez D, Revilla Y. Mechanisms of entry and endosomal pathway of African swine fever virus. Vaccines (Basel). 2017;5(4):42. doi: 10.3390/vaccines5040042. Epub 2017/11/09. PubMed PMID: 29117102; PubMed Central PMCID: PMC5748609.
- Alcami A, Carrascosa AL, Vinuela E. Interaction of African swine fever virus with macrophages. Virus Res. 1990;17(2):93–104. doi: 10.1016/0168-1702(90)90071-i. Epub 1990/10/01. PubMed PMID: 2291335.
- Alcami A, Carrascosa AL, Vinuela E. Saturable binding sites mediate the entry of African swine fever virus into vero cells. Virology. 1989;168(2):393–398. doi: 10.1016/0042-6822(89)90281-x. Epub 1989/02/01. PubMed PMID: 2916331.
- Carrascosa AL, Bustos MJ, Galindo I, et al. Virus-specific cell receptors are necessary, but not sufficient, to confer cell susceptibility to African swine fever virus. Arch Virol. 1999;144(7):1309–1321. doi: 10.1007/s007050050589. Epub 1999/09/11. PubMed PMID: 10481739.
- Sanchez-Torres C, Gomez-Puertas P, Gomez-Del-Moral M, et al. Expression of porcine CD163 on monocytes/macrophages correlates with permissiveness to African swine fever infection. Arch Virol. 2003;148(12):2307–2323. doi: 10.1007/s00705-003-0188-4. Epub 2003/12/04. PubMed PMID: 14648288.
- Popescu L, Gaudreault NN, Whitworth KM, et al. Genetically edited pigs lacking CD163 show no resistance following infection with the African swine fever virus isolate, Georgia 2007/1. Virology. 2017;501:102–106. doi: 10.1016/j.virol.2016.11.012. Epub 2016/11/30. PubMed PMID: 27898335.
- Lithgow P, Takamatsu H, Werling D, et al. Correlation of cell surface marker expression with African swine fever virus infection. Vet Microbiol. 2014;168(2–4):413–419. doi: 10.1016/j.vetmic.2013.12.001. Epub 2014/01/09. PubMed PMID: 24398227; PubMed Central PMCID: PMC3969584.
- Gao Q, Yang Y, Luo Y, et al. Adaptation of African swine fever virus to porcine kidney cells stably expressing CD163 and Siglec1. Front Immunol. 2022;13:1015224. doi: 10.3389/fimmu.2022.1015224. Epub 2022/11/18. PubMed PMID: 36389805; PubMed Central PMCID: PMC9647134.
- Pannhorst K, Carlson J, Hölper JE, et al. The non-classical major histocompatibility complex II protein SLA-DM is crucial for African swine fever virus replication. Sci Rep. 2023;13(1):10342. doi: 10.1038/s41598-023-36788-9. Epub 20230821. PubMed PMID: 37604847; PubMed Central PMCID: PMC10442341.
- Hernaez B, Alonso C. Dynamin- and clathrin-dependent endocytosis in African swine fever virus entry. J Virol. 2010;84(4):2100–2109. doi: 10.1128/JVI.01557-09. Epub 2009/11/27. PubMed PMID: 19939916; PubMed Central PMCID: PMC2812401.
- Chen X, Zheng J, Li T, et al. Coreceptor AXL facilitates African swine fever virus entry via apoptotic mimicry. J Virol. 2023;97(7):e0061623. doi: 10.1128/jvi.00616-23. Epub 20230629. PubMed PMID: 37382521; PubMed Central PMCID: PMC10373532.
- Sanchez EG, Quintas A, Perez-Nunez D, et al. African swine fever virus uses macropinocytosis to enter host cells. PLOS Pathog. 2012;8(6):e1002754. doi: 10.1371/journal.ppat.1002754. Epub 2012/06/22. PubMed PMID: 22719252; PubMed Central PMCID: PMC3375293.
- Basta S, Gerber H, Schaub A, et al. Cellular processes essential for African swine fever virus to infect and replicate in primary macrophages. Vet Microbiol. 2010;140(1–2):9–17. doi: 10.1016/j.vetmic.2009.07.015. Epub 2009/07/28. PubMed PMID: 19632793.
- Chen X, Zheng J, Liu C, et al. CD1d facilitates African swine fever virus entry into the host cells via clathrin-mediated endocytosis. Emerg Microbes Infect. 2023;12(2):2220575. doi: 10.1080/22221751.2023.2220575. PubMed PMID: 37254454; PubMed Central PMCID: PMC10288936.
- Andres G, Tsai B. African swine fever virus gets undressed: new insights on the entry pathway. J Virol. 2017;91(4). doi: 10.1128/JVI.01906-16. Epub 2016/12/16. PubMed PMID: 27974557; PubMed Central PMCID: PMC5286891.
- Colson P, De Lamballerie X, Yutin N, et al. “Megavirales”, a proposed new order for eukaryotic nucleocytoplasmic large DNA viruses. Arch Virol. 2013;158(12):2517–2521. doi: 10.1007/s00705-013-1768-6. Epub 2013/07/03. PubMed PMID: 23812617; PubMed Central PMCID: PMC4066373.
- Portugal R, Coelho J, Höper D, et al. Related strains of African swine fever virus with different virulence: genome comparison and analysis. J Gen Virol. 2015;96(2):408–419. doi: 10.1099/vir.0.070508-0. Epub 2014/11/20. PubMed PMID: 25406173.
- Yanez RJ, Rodrı́guez JM, Nogal ML, et al. Analysis of the complete nucleotide sequence of African swine fever virus. Virology. 1995;208(1):249–278. doi: 10.1006/viro.1995.1149. Epub 1995/04/01. PubMed PMID: 11831707.
- Chapman DAG, Tcherepanov V, Upton C, et al. Comparison of the genome sequences of non-pathogenic and pathogenic African swine fever virus isolates. J Gen Virol. 2008;89(2):397–408. doi: 10.1099/vir.0.83343-0. Epub 2008/01/17. PubMed PMID: 18198370.
- Zhenzhong W, Chuanxiang Q, Shengqiang G, et al. Genetic variation and evolution of attenuated African swine fever virus strain isolated in the field: a review. Virus Res. 2022;319:198874. doi: 10.1016/j.virusres.2022.198874. Epub 2022/07/26. PubMed PMID: 35872281.
- Mazloum A, van Schalkwyk A, Chernyshev R, et al. A guide to molecular characterization of genotype II African swine fever virus: essential and alternative genome markers. Microorganisms. 2023;11(3):642. doi: 10.3390/microorganisms11030642. Epub 2023/03/30. PubMed PMID: 36985215; PubMed Central PMCID: PMC10056344.
- Dinhobl M, Spinard E, Tesler N, et al. Reclassification of ASFV into 7 biotypes using unsupervised machine learning. Viruses. 2023;16(1):67. doi: 10.3390/v16010067. PubMed PMID.
- Spinard E, Rai A, Osei-Bonsu J, et al. The 2022 outbreaks of African swine fever virus demonstrate the first report of genotype II in Ghana. Viruses. 2023;15(8):1722. doi: 10.3390/v15081722. Epub 2023/08/26. PubMed PMID: 37632064; PubMed Central PMCID: PMC10459280.
- Zhao D, Sun E, Huang L, et al. Highly lethal genotype I and II recombinant African swine fever viruses detected in pigs. Nat Commun. 2023;14(1):3096. doi: 10.1038/s41467-023-38868-w. Epub 2023/05/30. PubMed PMID: 37248233; PubMed Central PMCID: PMC10226439.
- Spinard E, Dinhobl M, Tesler N, et al. A re-evaluation of African swine fever genotypes based on p72 sequences reveals the existence of only six distinct p72 groups. Viruses. 2023;15(11):2246. doi: 10.3390/v15112246. Epub 2023/11/25. PubMed PMID: 38005923; PubMed Central PMCID: PMC10675559.
- Portugal R, Coelho J, Hoper D, et al. Related strains of African swine fever virus with different virulence: genome comparison and analysis. J Gen Virol. 2015;96(Pt 2):408–419. doi: 10.1099/vir.0.070508-0. Epub 2014/11/20. PubMed PMID: 25406173.
- O’Donnell V, Holinka LG, Krug PW, et al. African swine fever virus Georgia 2007 with a deletion of virulence-associated gene 9GL (B119L), when administered at low doses, leads to virus attenuation in swine and induces an effective protection against homologous challenge. J Virol. 2015;89(16):8556–8566. doi: 10.1128/JVI.00969-15. Epub 2015/06/13. PubMed PMID: 26063424; PubMed Central PMCID: PMC4524225.
- Liu Y, Shen Z, Xie Z, et al. African swine fever virus I73R is a critical virulence-related gene: a potential target for attenuation. Proc Natl Acad Sci USA. 2023;120(15):e2210808120. doi: 10.1073/pnas.2210808120. Epub 2023/04/07. PubMed PMID: 37023125; PubMed Central PMCID: PMC10104517.
- Zhang K, Yang B, Shen C, et al. MGF360-9L is a major virulence factor associated with the African swine fever virus by antagonizing the JAK/STAT signaling pathway. MBio. 2022;13(1):e0233021. doi: 10.1128/mbio.02330-21. Epub 2022/01/26. PubMed PMID: 35076286; PubMed Central PMCID: PMC8788333.
- Yang J, Li S, Feng T, et al. African swine fever virus F317L protein inhibits NF-κB activation to evade host immune response and promote viral replication. mSphere. 2021;6(5):e0065821. doi: 10.1128/mSphere.00658-21. Epub 2021/10/21. PubMed PMID: 34668754; PubMed Central PMCID: PMC8527992.
- Rathakrishnan A, Connell S, Petrovan V, et al. Differential effect of deleting members of African swine fever virus multigene families 360 and 505 from the genotype II Georgia 2007/1 isolate on virus replication, virulence, and induction of protection. J Virol. 2022;96(6):e0189921. doi: 10.1128/jvi.01899-21. Epub 2022/01/20. PubMed PMID: 35044212; PubMed Central PMCID: PMC8941908.
- Abrams CC, Dixon LK. Sequential deletion of genes from the African swine fever virus genome using the cre/loxP recombination system. Virology. 2012;433(1):142–148. doi: 10.1016/j.virol.2012.07.021. Epub 2012/08/21. PubMed PMID: 22902236; PubMed Central PMCID: PMC3526793.
- Borca MV, Carrillo C, Zsak L, et al. Deletion of a CD2-like gene, 8-DR, from African swine fever virus affects viral infection in domestic swine. J Virol. 1998;72(4):2881–2889. Epub 1998/04/03. PubMed PMID: 9525608; PubMed Central PMCID: PMC109733
- O’Donnell V, Holinka LG, Gladue DP, et al. African swine fever virus Georgia isolate harboring deletions of MGF360 and MGF505 genes is attenuated in swine and confers protection against challenge with virulent parental virus. J Virol. 2015;89(11):6048–6056. doi: 10.1128/JVI.00554-15. Epub 2015/03/27. PubMed PMID: 25810553; PubMed Central PMCID: PMC4442422.
- Ramirez-Medina E, Vuono E, O’Donnell V, et al. Differential effect of the deletion of African swine fever virus virulence-associated genes in the induction of attenuation of the highly virulent Georgia strain. Viruses. 2019;11(7):599. doi: 10.3390/v11070599. Epub 2019/07/05. PubMed PMID: 31269702; PubMed Central PMCID: PMC6669436.
- Reis AL, Goatley LC, Jabbar T, et al. Deletion of the African swine fever virus gene DP148R does not reduce virus replication in culture but reduces virus virulence in pigs and induces high levels of protection against challenge. J Virol. 2017;91(24). doi: 10.1128/JVI.01428-17. Epub 2017/10/06. PubMed PMID: 28978700; PubMed Central PMCID: PMC5709585.
- Sanford B, Holinka LG, O’Donnell V, et al. Deletion of the thymidine kinase gene induces complete attenuation of the Georgia isolate of African swine fever virus. Virus Res. 2016;213:165–171. doi: 10.1016/j.virusres.2015.12.002. Epub 2015/12/15. PubMed PMID: 26656424.
- Ran Y, Li D, Xiong MG, et al. African swine fever virus I267L acts as an important virulence factor by inhibiting RNA polymerase III-RIG-I-mediated innate immunity. PLOS Pathog. 2022;18(1):e1010270. doi: 10.1371/journal.ppat.1010270. Epub 2022/01/29. PubMed PMID: 35089988; PubMed Central PMCID: PMC8827485.
- Ramirez-Medina E, Rai A, Espinoza N, et al. Deletion of the H240R gene in African swine fever virus partially reduces virus virulence in swine. Viruses. 2023;15(7):1477. doi: 10.3390/v15071477. Epub 2023/07/29.PubMed PMID: 37515164; PubMed Central PMCID: PMC10384018.
- Ramirez-Medina E, Vuono E, Rai A, et al. Deletion of E184L, a putative DIVA target from the pandemic strain of African swine fever virus, produces a reduction in virulence and protection against virulent challenge. J Virol. 2022;96(1):e0141921. doi: 10.1128/jvi.01419-21. Epub 2021/10/21. PubMed PMID: 34668772; PubMed Central PMCID: PMC8754217.
- Qi X, Feng T, Ma Z, et al. Deletion of DP148R, DP71L, and DP96R attenuates African swine fever virus, and the mutant strain confers complete protection against homologous challenges in pigs. J Virol. 2023;97(4):e0024723. doi: 10.1128/jvi.00247-23. Epub 2023/04/06. PubMed PMID: 37017515; PubMed Central PMCID: PMC10134827.
- Lopez E, Bosch-Camós L, Ramirez-Medina E, et al. Deletion mutants of the attenuated recombinant ASF virus, BA71ΔCD2, show decreased vaccine efficacy. Viruses. 2021;13(9):1678. doi: 10.3390/v13091678. Epub 2021/09/29. PubMed PMID: 34578263; PubMed Central PMCID: PMC8473413.
- Cadenas-Fernandez E, Sanchez-Vizcaino JM, Pintore A, et al. Free-ranging pig and wild boar interactions in an endemic area of African swine fever. Front Vet Sci. 2019;6:376. doi: 10.3389/fvets.2019.00376. Epub 2019/11/19. PubMed PMID: 31737649; PubMed Central PMCID: PMC6831522.
- Sauter-Louis C, Forth JH, Probst C, et al. Joining the club: first detection of African swine fever in wild boar in Germany. Transbound Emerg Dis. 2021;68(4):1744–1752. doi: 10.1111/tbed.13890. PubMed PMID: 33085828. Epub 2020/10/22.
- Migliore S, Hussein HA, Galluzzo P, et al. African swine fever and its control measures in wild boar: a “De Iure Condito” analysis in the European Union. Animals (Basel). 2023;14(1):14. doi: 10.3390/ani14010014. Epub 2024/01/11. PubMed PMID: 38200745; PubMed Central PMCID: PMC10778324.
- Dixon LK, Islam M, Nash R, et al. African swine fever virus evasion of host defences. Virus Res. 2019;266:25–33. doi: 10.1016/j.virusres.2019.04.002. Epub 2019/04/09. PubMed PMID: 30959069; PubMed Central PMCID: PMC6505686.
- Netherton CL, Connell S, Benfield CTO, et al. The genetics of life and death: virus-host interactions underpinning resistance to African swine fever, a viral hemorrhagic disease. Front Genet. 2019;10:402. doi: 10.3389/fgene.2019.00402. Epub 2019/05/28. PubMed PMID: 31130984; PubMed Central PMCID: PMC6509158.
- Penrith ML, Bastos AD, Etter EMC, et al. Epidemiology of African swine fever in Africa today: sylvatic cycle versus socio-economic imperatives. Transbound Emerg Dis. 2019;66(2):672–686. doi: 10.1111/tbed.13117. Epub 2019/01/12. PubMed PMID: 30633851.
- Thomson GR. The epidemiology of African swine fever: the role of free-living hosts in Africa. Onderstepoort J Vet Res. 1985;52(3):201–209. Epub 1985/09/01. PubMed PMID: 3911134.
- Thomson GR, Gainaru MD, Van Dellen AF. Experimental infection of warthogs (Phacochoerus aethiopicus) with African swine fever virus. Onderstepoort J Vet Res. 1980;47(1):19–22. Epub 1980/03/01. PubMed PMID: 7454231.
- Thomson GR, Gainaru MD, van Dellen AF. African swine fever: pathogenicity and immunogenicity of two non-haemadsorbing viruses. Onderstepoort J Vet Res. 1979;46(3):149–154. Epub 1979/09/01. PubMed PMID: 551362.
- Jori F, Vial L, Penrith ML, et al. Review of the sylvatic cycle of African swine fever in sub-Saharan Africa and the Indian ocean. Virus Res. 2013;173(1):212–227. doi: 10.1016/j.virusres.2012.10.005. Epub 2012/11/13. PubMed PMID: 23142551.
- Kukielka EA, Jori F, Martinez-Lopez B, et al. Wild and domestic pig interactions at the wildlife-livestock interface of Murchison falls national park, Uganda, and the potential association with African swine fever outbreaks. Front Vet Sci. 2016;3:31. doi: 10.3389/fvets.2016.00031. Epub 2016/05/06. PubMed PMID: 27148545; PubMed Central PMCID: PMC4831202.
- Heuschele WP, Coggins L. Isolation of African swine fever virus from a giant forest hog. Bull Epizoot Dis Afr. 1965;13(3):255–256. Epub 1965/09/01. PubMed PMID: 4283999.
- Sanchez-Botija C. African swine fever. New developments. Rev Scientifique et Technique. 1982;4:1065–1094.
- Plowright W, Parker J, Peirce MA. African swine fever virus in ticks (Ornithodoros moubata, murray) collected from animal burrows in Tanzania. Nature. 1969;221(5185):1071–1073. doi: 10.1038/2211071a0. Epub 1969/03/15. PubMed PMID: 5813153.
- Plowright W, Perry CT, Peirce MA. Transovarial infection with African swine fever virus in the argasid tick, Ornithodoros moubata porcinus, walton. Res Vet Sci. 1970;11(6):582–584. doi: 10.1016/S0034-5288(18)34259-0. Epub 1970/11/01. PubMed PMID: 5532269.
- Plowright W, Perry CT, Peirce MA. Experimental infection of the argasid tick, Ornithodoros moubata porcinus, with African swine fever virus. Arch Gesamte Virusforsch. 1970;31(1–2):33–50. doi: 10.1007/bf01241664. Epub 1970/01/01. PubMed PMID: 5475061.
- Forth JH, Forth LF, Lycett S, et al. Identification of African swine fever virus-like elements in the soft tick genome provides insights into the virus’ evolution. BMC Biol. 2020;18(1):136. doi: 10.1186/s12915-020-00865-6. Epub 2020/10/10. PubMed PMID: 33032594; PubMed Central PMCID: PMC7542975.
- García-Jiménez WL, Fernández-Llario P, Benítez-Medina JM, et al. Reducing Eurasian wild boar (Sus scrofa) population density as a measure for bovine tuberculosis control: effects in wild boar and a sympatric fallow deer (Dama dama) population in Central Spain. Prev Vet Med. 2013;110(3–4):435–446. doi: 10.1016/j.prevetmed.2013.02.017. Epub 2013/03/16. PubMed PMID: 23490145.
- Newsome T, Cairncross R, Cunningham CX, et al. Scavenging with invasive species. Biol Rev Camb Philos Soc. 2024;99(2):562–581. doi: 10.1111/brv.13035. Epub 2023/12/27. PubMed PMID: 38148253.
- Sanchez-Vizcaino JM, Mur L, Martinez-Lopez B. African swine fever (ASF): five years around Europe. Vet Microbiol. 2013;165(1–2):45–50. doi: 10.1016/j.vetmic.2012.11.030. Epub 2012/12/26. PubMed PMID: 23265248.
- Guinat C, Porphyre T, Gogin A, et al. Inferring within-herd transmission parameters for African swine fever virus using mortality data from outbreaks in the Russian Federation. Transbound Emerg Dis. 2018;65(2):e264–e271. doi: 10.1111/tbed.12748. Epub 2017/11/10. PubMed PMID: 29120101; PubMed Central PMCID: PMC5887875.
- Penrith ML, Vosloo W, Jori F, et al. African swine fever virus eradication in Africa. Virus Res. 2013;173(1):228–246. doi: 10.1016/j.virusres.2012.10.011. Epub 2012/11/13. PubMed PMID: 23142552.
- Zhou X, Li N, Luo Y, et al. Emergence of African swine fever in China, 2018. Transbound Emerg Dis. 2018;65(6):1482–1484. doi: 10.1111/tbed.12989. Epub 2018/08/14. PubMed PMID: 30102848.
- Gogin A, Gerasimov V, Malogolovkin A, et al. African swine fever in the North Caucasus region and the Russian federation in years 2007–2012. Virus Res. 2013;173(1):198–203. doi: 10.1016/j.virusres.2012.12.007. Epub 2012/12/26. PubMed PMID: 23266725.
- Carrasco L, Nunez A, Salguero FJ, et al. African swine fever: expression of interleukin-1 alpha and tumour necrosis factor-alpha by pulmonary intravascular macrophages. J Comp Pathol. 2002;126(2–3):194–201. doi: 10.1053/jcpa.2001.0543. Epub 2002/04/12. PubMed PMID: 11945008.
- Sierra MA, Carrasco L, Gomez-Villamandos JC, et al. Pulmonary intravascular macrophages in lungs of pigs inoculated with African swine fever virus of differing virulence. J Comput Pathol. 1990;102(3):323–334. doi: 10.1016/s0021-9975(08)80021-7. Epub 1990/04/01. PubMed PMID: 2365848.
- Mebus CA, Dardiri AH. Additional characteristics of disease caused by the African swine fever viruses isolated from Brazil and the Dominican Republic. Proc Annu Meet US Anim Health Assoc. 1979;1979(83):227–239. Epub 1979/01/01. PubMed PMID: 298918.
- Moulton J, Coggins L. Comparison of lesions in acute and chronic African swine fever. Cornell Vet. 1968;58(3):364–388. Epub 1968/07/01. PubMed PMID: 4297621.
- Carrasco L, Bautista MJ, Gomez-Villamandos JC, et al. Development of microscopic lesions in splenic cords of pigs infected with African swine fever virus. Vet Res. 1997;28(1):93–99. Epub 1997/01/01. PubMed PMID: 9172845.
- Konno S, Taylor WD, Hess WR, et al. Spleen pathology in African swine fever. Cornell Vet. 1972;62(3):486–506. Epub 1972/07/01. PubMed PMID: 5039613.
- Salguero FJ, Ruiz-Villamor E, Bautista MJ, et al. Changes in macrophages in spleen and lymph nodes during acute African swine fever: expression of cytokines. Vet Immunol Immunopathol. 2002;90(1–2):11–22. doi: 10.1016/s0165-2427(02)00225-8. Epub 2002/10/31. PubMed PMID: 12406651.
- Hervas J, Gomez-Villamandos JC, Mendez A, et al. Structural and ultrastructural study of glomerular changes in African swine fever. J Comput Pathol. 1996;115(1):61–75. doi: 10.1016/s0021-9975(96)80028-4. Epub 1996/07/01. PubMed PMID: 8878752.
- Hervas J, Gomez-Villamandos JC, Mendez A, et al. The lesional changes and pathogenesis in the kidney in African swine fever. Vet Res Commun. 1996;20(3):285–299. doi: 10.1007/bf00366926. Epub 1996/01/01. PubMed PMID: 8739527.
- Arias ML, Escribano JM, Rueda A, et al. La peste porcina africana. Med Veterinaire. 1986;3:333–350.
- Giammarioli M, Gallardo C, Oggiano A, et al. Genetic characterisation of African swine fever viruses from recent and historical outbreaks in Sardinia (1978–2009). Virus Genes. 2011;42(3):377–387. doi: 10.1007/s11262-011-0587-7. Epub 2011/03/05. PubMed PMID: 21373994.
- Goatley LC, Nash RH, Andrews C, et al. Cellular and humoral immune responses after immunisation with low virulent African swine fever virus in the large white inbred babraham line and outbred domestic pigs. Viruses. 2022;14(7):1487. doi: 10.3390/v14071487. Epub 2022/07/28. PubMed PMID: 35891467; PubMed Central PMCID: PMC9322176.
- Gallardo C, Soler A, Nieto R, et al. Experimental transmission of African swine fever (ASF) low virulent isolate NH/P68 by surviving pigs. Transbound Emerg Dis. 2015;62(6):612–622. doi: 10.1111/tbed.12431. Epub 2015/10/04. PubMed PMID: 26432068.
- Sánchez-Cordón PJ, Chapman D, Jabbar T, et al. Different routes and doses influence protection in pigs immunised with the naturally attenuated African swine fever virus isolate OURT88/3. Antiviral Res. 2017;138:1–8. doi: 10.1016/j.antiviral.2016.11.021. Epub 2016/12/03. PubMed PMID: 27908827; PubMed Central PMCID: PMC5245086.
- Martínez Avilés M, Bosch J, Ivorra B, et al. Epidemiological impacts of attenuated African swine fever virus circulating in wild boar populations. Research In Veterinary Science. 2023;162:104964. doi: 10.1016/j.rvsc.2023.104964. Epub 2023/08/03. PubMed PMID: 37531717.
- Fernandez de Marco M, Salguero FJ, Bautista MJ, et al. An immunohistochemical study of the tonsils in pigs with acute African swine fever virus infection. Res Vet Sci. 2007;83(2):198–203. doi: 10.1016/j.rvsc.2006.11.011. Epub 2007/01/30. PubMed PMID: 17258254.
- Gomez-Villamandos JC, Bautista MJ, Sanchez-Cordon PJ, et al. Pathology of African swine fever: the role of monocyte-macrophage. Virus Res. 2013;173(1):140–149. doi: 10.1016/j.virusres.2013.01.017. Epub 2013/02/05. PubMed PMID: 23376310.
- Gomez-Villamandos JC, Hervas J, Mendez A, et al. Experimental African swine fever: apoptosis of lymphocytes and virus replication in other cells. J Gen Virol. 1995;76(Pt 9):2399–2405. doi: 10.1099/0022-1317-76-9-2399. Epub 1995/09/01. PubMed PMID: 7561784.
- Salguero FJ, Sanchez-Cordon PJ, Nunez A, et al. Proinflammatory cytokines induce lymphocyte apoptosis in acute African swine fever infection. J Comput Pathol. 2005;132(4):289–302. doi: 10.1016/j.jcpa.2004.11.004. Epub 2005/05/17. PubMed PMID: 15893987.
- Salguero FJ, Sanchez-Cordon PJ, Sierra MA, et al. Apoptosis of thymocytes in experimental African swine fever virus infection. Histol Histopathol. 2004;19(1):77–84. doi: 10.14670/HH-19.77. Epub 2004/01/01. PubMed PMID: 14702174.
- Carbonnelle C, Moroso M, Pannetier D, et al. Natural history of Sudan ebolavirus to support medical countermeasure development. Vaccines (Basel). 2022;10(6):963. doi: 10.3390/vaccines10060963. Epub 2022/06/25. PubMed PMID: 35746571; PubMed Central PMCID: PMC9228702.
- Smither SJ, Nelson M, Eastaugh L, et al. Experimental respiratory Marburg virus haemorrhagic fever infection in the common marmoset (Callithrix jacchus). Int J Exp Pathol. 2013;94(2):156–168. doi: 10.1111/iep.12018. Epub 2013/02/28. PubMed PMID: 23441639; PubMed Central PMCID: PMC3607144.
- Smither SJ, Nelson M, Eastaugh L, et al. Experimental respiratory infection of marmosets (Callithrix jacchus) with Ebola virus kikwit. J Infect Dis. 2015;212(Suppl 2):S336–45. doi: 10.1093/infdis/jiv371. Epub 2015/07/26. PubMed PMID: 26209682.
- Watson RJ, Tree J, Fotheringham SA, et al. Dose-dependent response to infection with Ebola virus in the ferret model and evidence of viral evolution in the eye. J Virol. 2021;95(24):e0083321. doi: 10.1128/jvi.00833-21. Epub 2021/09/30. PubMed PMID: 34586862; PubMed Central PMCID: PMC8610581.
- Sanchez-Vizcaino JM, Slauson DO, Ruiz-Gonzalvo F, et al. Lymphocyte function and cell-mediated immunity in pigs with experimentally induced African swine fever. Am J Vet Res. 1981;42(8):1335–1341 Epub 1981/08/01. PubMed PMID: 6975049.
- Blome S, Gabriel C, Beer M. Pathogenesis of African swine fever in domestic pigs and European wild boar. Virus Res. 2013;173(1):122–130. doi: 10.1016/j.virusres.2012.10.026. Epub 2012/11/10. PubMed PMID: 23137735.
- Greig A. Pathogenesis of African swine fever in pigs naturally exposed to the disease. J Comput Pathol. 1972;82(1):73–79. doi: 10.1016/0021-9975(72)90028-x. Epub 1972/01/01. PubMed PMID: 4553010.
- Colgrove GS, Haelterman EO, Coggins L. Pathogenesis of African swine fever in young pigs. Am J Vet Res. 1969;30(8):1343–1359 Epub 1969/08/01. PubMed PMID: 4894999.
- Heuschele WP. Studies on the pathogenesis of African swine fever I. Quantitative studies on the sequential development of virus in pig tissues. Arch Gesamte Virusforsch. 1967;21(3–4):349–356. doi: 10.1007/bf01241735. Epub 1967/01/01. PubMed PMID: 4300741.
- Villeda CJ, Williams SM, Wilkinson PJ, et al. Consumption coagulopathy associated with shock in acute African swine fever. Arch Virol. 1993;133(3–4):467–475. doi: 10.1007/bf01313784. Epub 1993/01/01. PubMed PMID: 8257301.
- Mebus CA. African swine fever. Adv Virus Res. 1988;35:251–269. doi: 10.1016/s0065-3527(08)60714-9. Epub 1988/01/01. PubMed PMID: 3068966.
- Ramiro-Ibanez F, Ortega A, Escribano JM, et al. Apoptosis: a mechanism of cell killing and lymphoid organ impairment during acute African swine fever virus infection. J Gen Virol. 1996;77(9):2209–2219. doi: 10.1099/0022-1317-77-9-2209. Epub 1996/09/01. PubMed PMID: 8811021.
- Sierra MA, Quezada M, Fernandez A, et al. Experimental African swine fever: evidence of the virus in interstitial tissues of the kidney. Vet Pathol. 1989;26(2):173–176. doi: 10.1177/030098588902600211. Epub 1989/03/01. PubMed PMID: 2711573.
- Oura CA, Powell PP, Parkhouse RM. African swine fever: a disease characterized by apoptosis. J Gen Virol. 1998;79(6):1427–1438. doi: 10.1099/0022-1317-79-6-1427. Epub 1998/06/20. PubMed PMID: 9634085.
- Gomez Del Moral M, Ortuno E, Fernandez-Zapatero P, et al. African swine fever virus infection induces tumor necrosis factor alpha production: implications in pathogenesis. J Virol. 1999;73(3):2173–2180. doi: 10.1128/JVI.73.3.2173-2180.1999. Epub 1999/02/11. PubMed PMID: 9971800; PubMed Central PMCID: PMC104462.
- Franzoni G, Pedrera M, Sánchez-Cordón PJ. African swine fever virus infection and cytokine response in vivo: an update. Viruses. 2023;15(1):233. doi: 10.3390/v15010233. Epub 2023/01/22. PubMed PMID: 36680273; PubMed Central PMCID: PMC9864779.
- Zhang F, Hopwood P, Abrams CC, et al. Macrophage transcriptional responses following in vitro infection with a highly virulent African swine fever virus isolate. J Virol. 2006;80(21):10514–10521. doi: 10.1128/JVI.00485-06. Epub 2006/10/17. PubMed PMID: 17041222; PubMed Central PMCID: PMC1641748.
- Salguero FJ, White AD, Slack GS, et al. Comparison of rhesus and cynomolgus macaques as an infection model for COVID-19. Nat Commun. 2021;12(1):1260. doi: 10.1038/s41467-021-21389-9. Epub 2021/02/26. PubMed PMID: 33627662; PubMed Central PMCID: PMC7904795.
- Hu B, Huang S, Yin L. The cytokine storm and COVID-19. J med virol. 2021;93(1):250–256. doi: 10.1002/jmv.26232. Epub 2020/06/28. PubMed PMID: 32592501; PubMed Central PMCID: PMC7361342.
- Zheng Y, Li S, Li SH, et al. Transcriptome profiling in swine macrophages infected with African swine fever virus at single-cell resolution. Proc Natl Acad Sci USA. 2022;119(19):e2201288119. doi: 10.1073/pnas.2201288119. Epub 2022/05/05. PubMed PMID: 35507870; PubMed Central PMCID: PMC9171760.
- Carrasco L, Bautista MJ, Martin de las Mulas J, et al. Description of a new population of fixed macrophages in the splenic cords of pigs. J Anat. 1995;187(Pt 2):395–402.
- Gomez-Villamandos JC, Bautista MJ, Hervas J, et al. Subcellular changes in platelets in acute and subacute African swine fever. J Comput Pathol. 1996;115(4):327–341. doi: 10.1016/s0021-9975(96)80069-7. Epub 1996/11/01. PubMed PMID: 9004076.
- Gomez-Villamandos JC, Hervas J, Mendez A, et al. Ultrastructural study of the renal tubular system in acute experimental African swine fever: virus replication in glomerular mesangial cells and in the collecting ducts. Arch Virol. 1995;140(3):581–589. doi: 10.1007/bf01718433. Epub 1995/01/01. PubMed PMID: 7733828.
- Villeda CJ, Gomez-Villamandos JC, Williams SM, et al. The role of fibrinolysis in the pathogenesis of the haemorrhagic syndrome produced by virulent isolates of African swine fever virus. Thromb Haemost. 1995;73(1):112–117. doi: 10.1055/s-0038-1653734. Epub 1995/01/01. PubMed PMID: 7740481
- Bautista MJ, Gomez-Villamandos JC, Carrasco L, et al. Ultrastructural pathology of the bone marrow in pigs inoculated with a moderately virulent strain (DR’78) of African swine fever virus. Histol Histopathol. 1998;13(3):713–720. doi: 10.14670/HH-13.713. Epub 1998/08/05. PubMed PMID: 9690128.
- Gomez-Villamandos JC, Salguero FJ, Ruiz-Villamor E, et al. Classical swine fever: pathology of bone marrow. Vet Pathol. 2003;40(2):157–163. doi: 10.1354/vp.40-2-157. Epub 2003/03/15. PubMed PMID: 12637755.
- Carrasco L, de Lara FC, Gómez-Villamandos JC, et al. The pathogenic role of pulmonary intravascular macrophages in acute African swine fever. Res Vet Sci. 1996;61(3):193–198. doi: 10.1016/s0034-5288(96)90062-4. Epub 1996/11/01. PubMed PMID: 8938846.
- Carrasco L, Núñez A, Salguero FJ, et al. African swine fever: expression of interleukin-1 alpha and tumour necrosis factor-alpha by pulmonary intravascular macrophages. J Comp Pathol. 2002;126(2–3):194–201. doi: 10.1053/jcpa.2001.0543. Epub 2002/04/12. PubMed PMID: 11945008.
- Konno S, Taylor WD, Hess WR, et al. Liver pathology in African swine fever. Cornell Vet. 1971;61(1):125–150. Epub 1971/01/01. PubMed PMID: 5540970.
- Sanchez-Cordon PJ, Romero-Trevejo JL, Pedrera M, et al. Role of hepatic macrophages during the viral haemorrhagic fever induced by African swine fever virus. Histol Histopathol. 2008;23(6):683–691. doi: 10.14670/HH-23.683. Epub 2008/03/28. PubMed PMID: 18366006.
- Schäfer A, Franzoni G, Netherton CL, et al. Adaptive cellular immunity against African swine fever virus infections. Pathogens. 2022;11(2):274. doi: 10.3390/pathogens11020274. PubMed PMID
- Schäfer A, Hühr J, Schwaiger T, et al. Porcine invariant natural killer T cells: functional profiling and dynamics in steady state and viral infections. Front Immunol. 2019;10:1380. doi: 10.3389/fimmu.2019.01380. Epub 2019/07/19. PubMed PMID: 31316500; PubMed Central PMCID: PMC6611438.
- Takamatsu HH, Denyer MS, Lacasta A, et al. Cellular immunity in ASFV responses. Virus Res. 2013;173(1):110–121. doi: 10.1016/j.virusres.2012.11.009. Epub 2012/12/04. PubMed PMID: 23201582.
- Gómez-Villamandos JC, Carrasco L, Bautista MJ, et al. African swine fever and classical swine fever: a review of the pathogenesis. Dtsch Tierarztl Wochenschr. 2003;110(4):165–169. Epub 2003/05/22. PubMed PMID: 12756959.
- Sánchez-Cordón PJ, Núñez A, Salguero FJ, et al. Lymphocyte apoptosis and thrombocytopenia in spleen during classical swine fever: role of macrophages and cytokines. Vet Pathol. 2005;42(4):477–488. doi: 10.1354/vp.42-4-477. Epub 2005/07/12. PubMed PMID: 16006607.
- Sánchez-Cordón PJ, Romanini S, Salguero FJ, et al. Apoptosis of thymocytes related to cytokine expression in experimental classical swine fever. J Comput Pathol. 2002;127(4):239–248. doi: 10.1053/jcpa.2002.0587. Epub 2002/11/22. PubMed PMID: 12443731.
- Gómez-Villamandos JC, Ruiz-Villamor E, Bautista MJ, et al. Pathogenesis of classical swine fever: renal haemorrhages and erythrodiapedesis. J Comput Pathol. 2000;123(1):47–54. doi: 10.1053/jcpa.2000.0385. Epub 2000/07/25. PubMed PMID: 10906255.
- Everett H, Salguero FJ, Graham SP, et al. Characterisation of experimental infections of domestic pigs with genotype 2.1 and 3.3 isolates of classical swine fever virus. Vet Microbiol. 2010;142(1–2):26–33. doi: 10.1016/j.vetmic.2009.09.039. Epub 2009/10/31. PubMed PMID: 19875252.
- An TQ. Highly pathogenic porcine reproductive and respiratory syndrome virus, Asia. Emerg Infect Dis. 2011;17(9):1782–1784. doi: 10.3201/eid1709.110411. Epub 2011/09/06. PubMed PMID: 21888830; PubMed Central PMCID: PMC3322091.
- Morgan SB, Graham SP, Salguero FJ, et al. Increased pathogenicity of European porcine reproductive and respiratory syndrome virus is associated with enhanced adaptive responses and viral clearance. Vet Microbiol. 2013;163(1–2):13–22. doi: 10.1016/j.vetmic.2012.11.024. Epub 2013/01/15. PubMed PMID: 23313323.
- Morgan SB, Frossard JP, Pallares FJ, et al. Pathology and virus distribution in the lung and lymphoid tissues of pigs experimentally inoculated with three distinct type 1 PRRS virus isolates of varying pathogenicity. Transbound Emerg Dis. 2016;63(3):285–295. doi: 10.1111/tbed.12272. Epub 20141110. PubMed PMID: 25382098.
- Breese SS, Hess WR. Electron microscopy of African swine fever virus hemadsorption. J Bacteriol. 1966;92(1):272–274. doi: 10.1128/jb.92.1.272-274.1966. Epub 1966/07/01. PubMed PMID: 5949565; PubMed Central PMCID: PMC276226.
- Coggins L. A modified hemadsorption-inhibition test for African swine fever virus. Bull Epizoot Dis Afr. 1968;16(1):61–64. Epub 1968/03/01. PubMed PMID: 5693874.
- Malmquist WA. Propagation, modification, and hemadsorption of African swine fever virus in cell cultures. Am J Vet Res. 1962;23:241–247. Epub 1962/03/01. PubMed PMID: 14469017.
- Lim JW, Vu TTH, Le VP, et al. Advanced strategies for developing vaccines and diagnostic tools for African swine fever. Viruses. 2023;15(11):2169. doi: 10.3390/v15112169. Epub 2023/11/25. PubMed PMID: 38005846; PubMed Central PMCID: PMC10674204.
- Fernández-Pinero J, Gallardo C, Elizalde M, et al. Molecular diagnosis of African swine fever by a new real-time PCR using universal probe library. Transbound Emerg Dis. 2013;60(1):48–58. doi: 10.1111/j.1865-1682.2012.01317.x. Epub 2012/03/08. PubMed PMID: 22394449.
- King DP, Reid SM, Hutchings GH, et al. Development of a TaqMan PCR assay with internal amplification control for the detection of African swine fever virus. J Virol Methods. 2003;107(1):53–61. doi: 10.1016/s0166-0934(02)00189-1. Epub 2002/11/26. PubMed PMID: 12445938.
- Agüero M, Fernández J, Romero L, et al. Highly sensitive PCR assay for routine diagnosis of African swine fever virus in clinical samples. J Clin Microbiol. 2003;41(9):4431–4434. doi: 10.1128/jcm.41.9.4431-4434.2003. Epub 2003/09/06. PubMed PMID: 12958285; PubMed Central PMCID: PMC193827.
- Zhao Y, Chen F, Li Q, et al. Isothermal amplification of nucleic acids. Chem Rev. 2015;115(22):12491–12545. doi: 10.1021/acs.chemrev.5b00428. Epub 2015/11/10. PubMed PMID: 26551336.
- Reid MS, Le XC, Zhang H. Exponential isothermal amplification of nucleic acids and assays for proteins, cells, small molecules, and enzyme activities: an EXPAR example. Angew Chem (Int Ed In English). 2018;57(37):11856–11866. doi: 10.1002/anie.201712217. Epub 2018/04/29. PubMed PMID: 29704305.
- Pumford EA, Lu J, Spaczai I, et al. Developments in integrating nucleic acid isothermal amplification and detection systems for point-of-care diagnostics. Biosens Bioelectron. 2020;170:112674. doi: 10.1016/j.bios.2020.112674. Epub 2020/10/10. PubMed PMID: 33035900; PubMed Central PMCID: PMC7529604.
- Notomi T, Mori Y, Tomita N, et al. Loop-mediated isothermal amplification (LAMP): principle, features, and future prospects. J Microbiol. 2015;53(1):1–5. doi: 10.1007/s12275-015-4656-9. Epub 2015/01/06. PubMed PMID: 25557475.
- Lee H, Lee S, Park C, et al. Rapid visible detection of African swine fever virus using hybridization chain reaction-sensitized magnetic nanoclusters and affinity chromatography. Small. 2023;19(26):e2207117. doi: 10.1002/smll.202207117. Epub 2023/03/25. PubMed PMID: 36960666.
- James HE, Ebert K, McGonigle R, et al. Detection of African swine fever virus by loop-mediated isothermal amplification. J Virol Methods. 2010;164(1–2):68–74. doi: 10.1016/j.jviromet.2009.11.034. Epub 2009/12/08. PubMed PMID: 19963011.
- Fan X, Li L, Zhao Y, et al. Clinical validation of two recombinase-based isothermal amplification assays (RPA/RAA) for the rapid detection of African swine fever virus. Front Microbiol. 2020;11:1696. doi: 10.3389/fmicb.2020.01696. Epub 2020/08/15. PubMed PMID: 32793160; PubMed Central PMCID: PMC7385304.
- Qian S, Chen Y, Peng C, et al. Dipstick-based rapid nucleic acids purification and CRISPR/Cas12a-mediated isothermal amplification for visual detection of African swine fever virus. Talanta. 2022;242:123294. doi: 10.1016/j.talanta.2022.123294. Epub 2022/02/13. PubMed PMID: 35149424.
- Giménez-Lirola LG, Mur L, Rivera B, et al. Detection of African swine fever virus antibodies in serum and oral fluid specimens using a recombinant protein 30 (p30) dual matrix indirect ELISA. PLOS ONE. 2016;11(9):e0161230. doi: 10.1371/journal.pone.0161230. Epub 2016/09/10. PubMed PMID: 27611939; PubMed Central PMCID: PMC5017782 the research authorship, and/or publication of this article: authors S. Lizano and C. Goodell are employed by IDEXX Laboratories, Inc. The remaining authors declare no conflicting interests with respect to their authorship or the publication of this article. The commercial affiliation above mentioned does not alter the authors’ adherence to PLOS ONE policies on sharing data and materials.
- Aira C, Ruiz T, Dixon L, et al. Bead-based multiplex assay for the simultaneous detection of antibodies to African swine fever virus and classical swine fever virus. Front Vet Sci. 2019;6:306. doi: 10.3389/fvets.2019.00306. Epub 2019/10/02. PubMed PMID: 31572739; PubMed Central PMCID: PMC6753221.
- Wan Y, Shi Z, Peng G, et al. Development and application of a colloidal-gold dual immunochromatography strip for detecting African swine fever virus antibodies. Appl Microbiol Biotechnol. 2022;106(2):799–810. doi: 10.1007/s00253-021-11706-z. Epub 2021/12/24. PubMed PMID: 34939134.
- Mur L, Martínez-López B, Martínez-Avilés M, et al. Quantitative risk assessment for the introduction of African swine fever virus into the European Union by legal import of live pigs. Transbound Emerg Dis. 2012;59(2):134–144. doi: 10.1111/j.1865-1682.2011.01253.x. Epub 2011/08/13. PubMed PMID: 21831148.
- Mur L, Martínez-López B, Sánchez-Vizcaíno JM. Risk of African swine fever introduction into the European Union through transport-associated routes: returning trucks and waste from international ships and planes. BMC Vet Res. 2012;8(1):149. doi: 10.1186/1746-6148-8-149. Epub 2012/09/01. PubMed PMID: 22935221; PubMed Central PMCID: PMC3485109.
- Mur L, Boadella M, Martínez-López B, et al. Monitoring of African swine fever in the wild boar population of the most recent endemic area of Spain. Transbound Emerg Dis. 2012;59(6):526–531. doi: 10.1111/j.1865-1682.2012.01308.x. Epub 2012/01/18. PubMed PMID: 22248024.
- Arias M, Jurado C, Gallardo C, et al. Gaps in African swine fever: analysis and priorities. Transbound Emerg Dis. 2018;65(S1):235–247. doi: 10.1111/tbed.12695.
- WOAH. African swine fever: WOAH warns Veterinary Authorities and pig industry of risk from use of sub-standard vaccines. 2023. https://www.woah.org/en/african-swine-fever-woah-warns-veterinary-authorities-and-pig-industry-of-risk-from-use-of-sub-standard-vaccines/
- Argilaguet JM, Perez-Martin E, Lopez S, et al. BacMam immunization partially protects pigs against sublethal challenge with African swine fever virus. Antiviral Res. 2013;98(1):61–65. doi: 10.1016/j.antiviral.2013.02.005. Epub 2013/02/23. PubMed PMID: 23428670.
- Argilaguet JM, Perez-Martin E, Nofrarias M, et al. DNA vaccination partially protects against African swine fever virus lethal challenge in the absence of antibodies. PLOS ONE. 2012;7(9):e40942. doi: 10.1371/journal.pone.0040942. Epub 2012/10/11. PubMed PMID: 23049728; PubMed Central PMCID: PMC3458849.
- Lacasta A, Ballester M, Monteagudo PL, et al. Expression library immunization can confer protection against lethal challenge with African swine fever virus. J Virol. 2014;88(22):13322–13332. doi: 10.1128/JVI.01893-14. Epub 2014/09/12. PubMed PMID: 25210179; PubMed Central PMCID: PMC4249112.
- Jancovich JK, Chapman D, Hansen DT, et al. Immunization of pigs by DNA prime and recombinant vaccinia virus boost to identify and rank African swine fever virus immunogenic and protective proteins. J Virol. 2018;92(8). doi: 10.1128/JVI.02219-17. Epub 2018/02/02. PubMed PMID: 29386289; PubMed Central PMCID: PMC5874426.
- Argilaguet J, Pérez-Martín E, Gallardo C, et al. Enhancing DNA immunization by targeting ASFV antigens to SLA-II bearing cells. Vaccine. 2011;29(33):5379–5385. doi: 10.1016/j.vaccine.2011.05.084.
- Goatley LC, Reis AL, Portugal R, et al. A pool of eight virally vectored African swine fever antigens protect pigs against fatal disease. Vaccines. 2020;8(2):234. doi: 10.3390/vaccines8020234.
- Chen W, Zhao D, He X, et al. A seven-gene-deleted African swine fever virus is safe and effective as a live attenuated vaccine in pigs. Sci China Life Sci. 2020;63(5):623–634. doi: 10.1007/s11427-020-1657-9. Epub 2020/03/04. PubMed PMID: 32124180; PubMed Central PMCID: PMC7223596.
- Deutschmann P, Forth JH, Sehl-Ewert J, et al. Assessment of African swine fever vaccine candidate ASFV-G-∆MGF in a reversion to virulence study. NPJ Vaccines. 2023;8(1):78. doi: 10.1038/s41541-023-00669-z. Epub 2023/05/30. PubMed PMID: 37248243; PubMed Central PMCID: PMC10227017 Zoetis has a commercial license for the vaccine candidate described in the manuscript. The overall project, coordinated by Sandra Blome at the FLI, received funding by Zoetis, including the salary of Paul Deutschmann. All other authors declare no competing interests.
- Sánchez-Botija CJRo AP, Biology. Modificaciones del virus de la PPA en cultivos celulares. Patogenicidad y propiedades protectivas de las cepas atenuadas. 1963;7:5–23.
- Manso-Ribeiro J, Nunes-Petisca J, Lopez-Frazao F, et al. Vaccination Against ASF. 1963;60:921–937.
- Ramirez-Medina E, Velazquez-Salinas L, Rai A, et al. Evaluation of the deletion of the African swine fever virus gene O174L from the genome of the Georgia isolate. Viruses. 2023;15(10):2134. doi: 10.3390/v15102134. Epub 2023/10/28. PubMed PMID: 37896911; PubMed Central PMCID: PMC10612027.
- Boinas F, Hutchings G, Dixon L, et al. Characterization of pathogenic and non-pathogenic African swine fever virus isolates from Ornithodoros erraticus inhabiting pig premises in Portugal. Journal of General Virology. 2004;85(8):2177–2187. doi: 10.1099/vir.0.80058-0. PMID: 15269356.
- Gallardo C, Soler A, Nieto R, Mur L, Pérez C, Pelayo V, editors. Protection of European domestic pigs from Armenia virulent African swine fever virus by experimental immunisation using the attenuated and non-haemadsorbing African swine fever virus isolate ASFV/NH/P68. Proceedings of the 9th Annual ESVV Congress Madrid. Madrid (Spain); 2012.
- King K, Chapman D, Argilaguet JM, et al. Protection of European domestic pigs from virulent African isolates of African swine fever virus by experimental immunisation. Vaccine. 2011;29(28):4593–4600. doi: 10.1016/j.vaccine.2011.04.052.
- Leitão A, Cartaxeiro C, Coelho R, et al. The non-haemadsorbing African swine fever virus isolate ASFV/NH/P68 provides a model for defining the protective anti-virus immune response. J Gen Virol. 2001;82(3):513–523. doi: 10.1099/0022-1317-82-3-513.